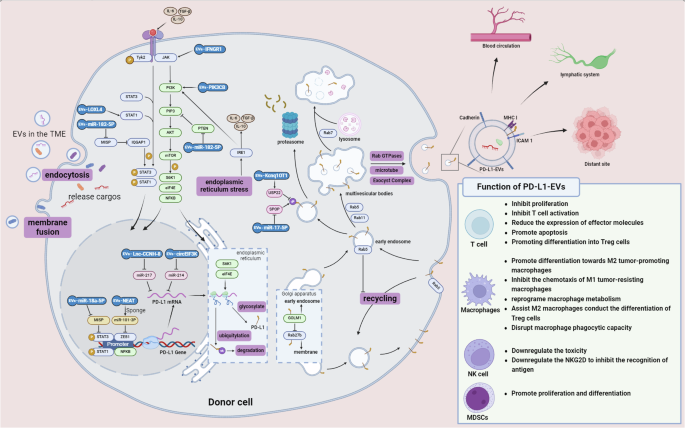
- Select a language for the TTS:
- UK English Female
- UK English Male
- US English Female
- US English Male
- Australian Female
- Australian Male
- Language selected: (auto detect) - EN
Play all audios:
ABSTRACT Immune checkpoint proteins (ICPs) serve as critical regulators of the immune system, ensuring protection against damage due to overly activated immune responses. However, within the
tumor environment, excessive ICP activation weakens antitumor immunity. Despite the development of numerous immune checkpoint blockade (ICB) drugs in recent years, their broad application
has been inhibited by uncertainties about their clinical efficacy. A thorough understanding of ICP regulation in the tumor microenvironment is essential for advancing the development of more
effective and safer ICB therapies. Extracellular vesicles (EVs), which are pivotal mediators of cell–cell communication, have been extensively studied and found to play key roles in the
functionality of ICPs. Nonetheless, a comprehensive review summarizing the current knowledge about the crosstalk between EVs and ICPs in the tumor environment is lacking. In this review, we
summarize the interactions between EVs and several widely studied ICPs as well as their potential clinical implications, providing a theoretical basis for further investigation of EV-related
ICB therapeutic approaches. SIMILAR CONTENT BEING VIEWED BY OTHERS PD-1/CD80+ SMALL EXTRACELLULAR VESICLES FROM IMMUNOCYTES INDUCE COLD TUMOURS FEATURED WITH ENHANCED ADAPTIVE
IMMUNOSUPPRESSION Article Open access 08 May 2024 EXTRACELLULAR VESICLES IN IMMUNOMODULATION AND TUMOR PROGRESSION Article 22 March 2021 TUMOUR-INTRINSIC ENDOMEMBRANE TRAFFICKING BY ARF6
SHAPES AN IMMUNOSUPPRESSIVE MICROENVIRONMENT THAT DRIVES MELANOMAGENESIS AND RESPONSE TO CHECKPOINT BLOCKADE THERAPY Article Open access 04 August 2024 INTRODUCTION The discovery of CTLA4 as
a pivotal inhibitor of the immune response in 1991 marked the beginning of extensive research in immune checkpoint proteins (ICPs)1, leading to the identification of various members of this
large family, which includes inhibitors such as programmed death-ligand 1 (PD-L1), cytotoxic T-lymphocyte antigen 4 (CTLA4), lymphocyte-activation gene 3 (LAG3), T-cell immunoglobulin and
mucin-domain containing-3 (TIM3) as well as stimulators such as inducible T-cell CO-stimulator (ICOS)2,3. These receptors and ligands play crucial roles in regulating immune cell functions
and maintaining immune homeostasis4. However, in cancer, these checkpoints are often overactivated, leading to impaired antitumor immunity5. Inhibiting the activation of ICPs to restore
immune cell function has been utilized in the clinical treatment of cancer; for example, in 2011, the Food and Drug Administration (FDA) authorized ipilimumab for the treatment of advanced
unresectable melanoma6,7. Nonetheless, researchers acknowledge current issues with immune checkpoint-related therapies, such as variability in patient benefits, resistance in some patients,
and the nonnegligible incidence of severe autoimmune symptoms8,9. Therefore, the exploration of safer and more effective ICB therapies is urgently needed. This requires a deeper
understanding of the mechanisms of action of ICPs. Current studies posit that ICPs contribute to tumor immune evasion via the downstream signals they mediate. The processes of their
expression, transport, activation, and degradation are influenced by a plethora of ‘messengers’ within the tumor microenvironment (TME)3,10,11. EVs are phospholipid membrane-coated particles
that selectively carry cytoplasmic components, such as proteins, RNA, and lipids, derived from donor cells, and EVs are considered potentially the most complex form of intercellular
communication12. According to the Minimal Information for Studies of Extracellular Vesicles (MISEV) guidelines that were introduced by the International Society for Extracellular Vesicles
(ISEV) in 2014 and updated in 2018, EVs can be classified from multiple dimensions. Based on their biogenesis, EVs can be classified as exosomes derived from endosomes or microvesicles
originating from the plasma membrane13. EVs are believed to play significant roles in promoting tumor cell proliferation, angiogenesis, metastasis, immune suppression, and drug
tolerance14,15. In particular, the cargoes that are carried by EVs have been found to exert profound regulatory effects on the activation, proliferation, secretion, and apoptosis of various
immune cells11,16,17,18. Moreover, extensive researches have shown that these functions can be achieved by affecting ICPs on target cells (Table 1). ICP-related EVs also demonstrate immense
potential in tumor therapy; for example, EVs carrying ICPs can be used to monitor the response to ICB therapy and the prognosis of patients with tumors19,20,21, and certain cargoes within
EVs have been found to be capable of downregulating ICPs on immune cell surfaces, thereby facilitating tumor immunity or enhancing ICB therapeutic efficacy22,23,24. Given the pivotal roles
of EVs in the spectrum of ICP functions, in order to increase the efficacy and safety of ICB therapies, further exploration of how EVs influence ICPs is crucial. In this review, we
comprehensively describe the interactions between EVs and major ICPs, elucidating how EVs influence the production and transportation of ICPs, as well as the roles of ICPs that are carried
by EVs. Additionally, we review the efforts made to apply ICP-related EVs in clinical practice. Our aim is to shed light on potential directions for future research on the link between EVs
and ICPs, offering insights for advancing tumor therapy. CROSSTALK BETWEEN EVS AND PD-L1 PD-L1 (B7-H1 or CD274) is a 290-amino acid protein that belongs to the type I transmembrane protein
receptor B7 family. It is expressed on the surfaces of various cell types, including antigen-presenting cells (APCs), T cells, B cells, monocytes, and epithelial cells25,26. When PD-L1
specifically binds to PD-1 on the surface of target cells, it inhibits downstream pathways related to immune cell activation and proliferation, such as the PI3K/AKT/mTOR and RAS/MEK/ERK1/2
pathways, by recruiting Src homology region 2-containing protein tyrosine phosphatase 2 (SHP2)3. Extensive researches have demonstrated a close association between PD-L1 and EVs; EVs can
increase the expression of PD-L1 and facilitate its intercellular transport, and in turn, PD-L1 is selectively packaged into EVs to exert its effects (Fig. 1). EVS REGULATE PD-L1 BIOGENESIS
Under the regulation of transcription factors and cis-acting elements, PD-L1 is expressed and then transported to the surface of the endoplasmic reticulum. After translation and
posttranslational modification, PD-L1 is delivered to the cell surface27. A significant portion of PD-L1 is subsequently loaded into EVs and released from the cell. The specific mechanisms
are detailed in Fig. 1. The regulators of PD-L1 transcription that are carried by EVs are diverse, and current studies have focused mainly on PI3K/Akt pathway components and signal
transducer and activator of transcription 1/3 (STAT1/3) proteins. The PI3K/Akt pathway, which is commonly hyperactivated in tumor cells, plays critical roles in cancer cell proliferation,
immune evasion, and drug resistance. It primarily activates PD-L1 transcription by phosphorylating the downstream transcription factor NFκB28. EVs from esophageal squamous cell carcinoma
cells carrying the PI3K catalytic subunit PIK3CB enters other tumor cells, promoting PD-L1 promoter transcriptional activity29. STAT1/3, which are downstream transcription factors of
interferon I, are also involved in EV regulation. EVs derived from various tumor cells carry protein or RNA cargo that promotes PD-L1 expression by increasing the phosphorylation of STAT1/3
or by assisting these transcriptional regulators in binding to the PD-L1 gene30,31. Additionally, under normal conditions, fibroblastic reticular cells directly interact with T cells in
lymph nodes, providing survival signals. EVs from head and neck squamous cell carcinoma cells transport phosphorylated interferon gamma receptor 1 (IFNGR1) to fibroblastic reticular cells
within sentinel lymph nodes, activating the JAK/STAT1 pathway without binding to IFNG, thereby promoting PD-L1 transcription32. The functional transformation of these EVs in the tumor
microenvironment indicates that the cargo that is carried by EVs can subvert the body’s antitumor immune response and create a favorable survival environment that facilitates tumor lymph
node metastasis via PD-L133. The regulation of PD-L1 transcription is not limited to these pathways. For example, EVs that are derived from M2 macrophages carry the long noncoding RNA NEAT1
sponge miR-101-3p, which upregulates the transcription factor zinc finger E-box-binding homeobox 1 (ZEB1), thereby promoting PD-L1 expression by tumor cells34,35. EV cargo can also target
microRNAs that directly act on PD-L1 mRNA. For example, EVs from hypoxic tumor-associated fibroblasts carry circEIF3K, which can enter tumor cells and downregulate the ability of miR-214 to
target PD-L136. EVs from hepatocellular carcinoma (HCC) cells carry Lnc-CCNH-8, which acts as a sponge for miR-21737. The unstable intracellular environment caused by active protein
synthesis in tumor cells, as well as the production of abundant reactive oxygen species (ROS) due to radiotherapy and chemotherapy, can lead to protein folding abnormalities in the
endoplasmic reticulum (ER), resulting in endoplasmic reticulum stress (ERS)38. Although excessive stress can induce apoptosis in tumor cells, in most cases, tumor cells restore intracellular
homeostasis by increasing ER efficiency and degrading abnormally folded proteins, thereby increasing survival39. EVs from head and neck squamous cell carcinoma cells undergoing ERS induce
macrophage M2 polarization by upregulating the PI3K/AKT pathway40. Subsequently, increased levels of IL-6 and IL-10 synergistically activate the ERS-related IRE1α–XBP1 signaling pathway,
which further contributes to the expression of inflammatory factors and promotes the expression of PD-L1 in M2 macrophages41. This finding was validated in another study42; EVs derived from
breast cancer cells undergoing ERS carry miR-27a-3p, which targets the ERS-related protein MAGI2 to downregulate PTEN, thereby promoting PD-L1 expression in M2 macrophages. Interestingly,
upon receiving endoplasmic reticulum stress signals, M2 macrophages experience lysosomal overload, leading to the potential extracellular release of numerous proteases, including cathepsins.
This can undermine the stroma’s ability to restrict the tumor42. Concurrently, this reduces the adhesion of EVs to the stroma, potentially allowing more EVs to diffuse to prospective
metastatic sites and assisting in tumor metastasis. The hypoxic tumor microenvironment prompts an increase in ROS, which can modify EV-mediated cellular communication, thereby modulating
PD-L1 expression. For example, the accumulation of ROS in the peritoneal metastases of ovarian cancer prevents M2 macrophages from absorbing tumor-derived EVs carrying miR-155-5p, preventing
the downregulation of PD-L143. Unfortunately, research on the role of EVs in the regulation of PD-1 transcription is limited. Yuan et al.44 reported that EVs from esophageal squamous cell
carcinoma stem cells carrying the nutrient sensor O-GlcNAc transferase (OGT) enter CD8+ T cells to promote PD-1 expression, indicating that EVs are indeed involved in the transcription of
PD-1, which may be a future research direction. Posttranscriptional regulation and posttranslational modification of PD-L1 are quality control measures for its intracellular transport and
are considered crucial mechanisms for tumor immune suppression45. Notably, the N-glycosylation of PD-L1 appears to be of paramount importance. Studies have indicated that N-glycosylation of
PD-L1 is directly related to its expression level on the cell surface, with glycosylation preventing the proteasomal degradation of PD-L1 after its interaction with glycogen synthase kinase
3β (GSK3β)46,47,48. Zhu et al.49,50 reported that the glycosylation of PD-L1 on the surface of EVs is a prerequisite for its binding and interaction with PD-1, making glycosylated PD-L1 on
patient EVs a more reliable potential tumor biomarker. The ubiquitination of PD-L1 mediates its proteasome-dependent degradation, and EVs in the tumor microenvironment also inhibit this
process, thereby increasing PD-L1 expression levels. Speckle-type POZ protein (SPOP) is one of the primary E3 ubiquitin ligases that mediates PD-L1 ubiquitination degradation, transferring
E2 ubiquitin-conjugating enzymes and E1 ubiquitin-activating enzymes to PD-L1. Colorectal cancer stem cell-derived EVs carry miR-17-5p to inhibit SPOP expression, thereby inhibiting
intracellular PD-L1 ubiquitination-mediated degradation51,52. Conversely, tumor cell-derived EVs carry the long noncoding RNA Kcnq1OT1, which competes with miR-30A-5p to bind to
ubiquitin-specific protease 22 (USP22), increasing USP22 expression and severing the link between PD-L1 and ubiquitin chains53. However, many questions remain regarding the role of EVs in
the ubiquitination of PD-L1, and there is a lack of research on whether EVs also participate in the ubiquitination-mediated degradation of PD-1. EVS CARRY PD-L1 INTO THE TUMOR
MICROENVIRONMENT PD-L1, which is a membrane-bound ligand, has been extensively studied and found to rely primarily on EVs for its intercellular transport54,55,56. PD-L1 on the plasma
membrane undergoes continuous endocytosis. Some internalized PD-L1 proteins return to the cell surface, whereas others are loaded into EVs along with endoplasmic reticulum proteins12,57. The
strong colocalization of PD-L1 with the EV marker CD63 within multivesicular body (MVB) substructures suggests that donor cells selectively load PD-L1 into EVs58. The sorting of PD-L1 to
the plasma membrane or early endosomes begins in the Golgi apparatus, where overexpressed Golgi membrane protein 1 (GOLM1) binds directly to PD-L1 for transport. Ras-associated proteins
(Rabs) are part of the Ras superfamily of small GTPases and play an important role in producing secretory forms of molecules within cells59; among them, Rab27b facilitates the transport of
PD-L1 to the cell surface, whereas GOLM1 downregulates Rab27b levels, restraining PD-L1 within the trans-Golgi network area and ultimately leading to its entry into early endosomes60. The
membranes of early endosomes invaginate to form MVBs, creating intraluminal vesicles (ILVs) that carry PD-L1. By increasing the efficiency of this process, donor cells can facilitate the
release of PD-L1-EVs. For example, Rab5 colocalizes with internalized PD-L1 on the cell membrane, aiding in its transport to MVBs and inhibiting its retransport back to the cell surface61.
The downregulation of histone lysine-specific demethylase 1 (LSD1) in gastric cancer cells reduces the number of MVBs and the secretion of EVs containing PD-L1, as does the downregulation of
Rab11, which facilitates MVB maturation62,63. Surprisingly, this also decreases the intracellular expression of TSG101, a component of the endosomal sorting complex required for transport,
which binds to the surface of MVBs to induce their degradation through lysosomes57,64; these findings suggest complex and but unknown regulatory mechanisms underlying the assembly of
PD-L1-containing EVs. ERS in donor cells can also promote the formation of MVBs. The expression levels of the ERS-related proteins inositol-requiring enzyme 1 (IRE1) and PKR-like ER kinase
(PERK) are increased in tumor cells, promoting the phosphorylation of proteins related to MVB formation65. Mature MVBs carrying PD-L1 can fuse with lysosomes for degradation or be
transported to the cell surface for release66. Fusion with lysosomes is dependent on Rab7, whereas transport to the cell surface has recently been linked to the exocyst complex, an octameric
protein complex that binds to phosphatidylinositol 4-phosphate (PI4P) and Rab11 on MVB surfaces. The Exo84 subunit of the exocyst complex then assembles with the Sec3 subunit on the inner
side of the plasma membrane, anchoring MVBs for release67. Additionally, the Exo70 subunit within this complex is also directly involved in the transport of MVBs to the plasma membrane68.
P53, the central regulator of the DNA damage response in tumor cells, is another factor that is closely associated with MVB release in response to environmental stress in tumor cells. P53
regulates the fusion of MVBs with the plasma membrane through the transmembrane protein TASP6, thereby increasing the number of EVs loaded with PD-L169,70. However, the high expression of
cytotoxin-associated gene A (CagA) in _Helicobacter pylori_-related gastric cancer cells has been found to increase PD-L1 content in EVs by downregulating P53, suggesting additional
regulatory functions of P53 in the content of PD-L1 in EVs71. The opening of calcium channels, specifically QRAI1, on the surface of lung cancer cells provides a stable calcium environment
for the function of Rab27a and synaptotagmin-like protein 2 (SLP2-a) during the fusion of MVBs containing PD-L1 with the plasma membrane55. In summary, tumor cells create an optimal
environment for selectively packaging PD-L1 into EVs, thereby facilitating its function. Notably, PD-1 is also internalized from the cell membrane, but very few studies have attempted to
explore whether it can be repackaged into EVs or to determine the regulatory role of donor cells in this process5,72. EVs in the tumor microenvironment, despite lacking active motility, can
accumulate at specific sites under the influence of adhesion-related proteins12. EVs carrying PD-L1 express fewer adhesion proteins that bind to fibronectin and collagen in the extracellular
matrix, which reduces their retention by the tumor extracellular matrix and allows rapid dispersion beyond the tumor tissue73. Conversely, these EVs express high levels of cadherins, which
are thought to mediate adhesion to various endothelial cells, ultimately guiding EVs to enter lymph nodes, the vascular system, and distant metastatic sites rich in endothelial cells, such
as lung tissue73,74,75. EVs carrying PD-L1 also express high levels of the intercellular adhesion molecule 1 (ICAM1) and MHC class I molecules, which, along with PD-L1, form immune synapses
to specifically bind to CD8+ T cells, thereby attacking T cells within lymph nodes73,76. The unique biological behavior exhibited by PD-L1-expressing EVs may represent a potential direction
for future targeted therapeutic approaches. PD-L1 EXERTS FUNCTIONS VIA EVS PD-1/PD-L1 signaling plays a critical role in shaping the immunosuppressive tumor microenvironment3,5. However, the
efficiency of signal transduction activated by cell surface PD-L1/PD-1 interactions is limited by the cell contact surface area. Consequently, cells within the tumor microenvironment
cleverly load PD-L1 onto EVs. This strategy not only provides more available PD-L1 binding sites on the target cell surface but also allows PD-L1 to exert its effects beyond the location of
the donor cell within the microenvironment73. Numerous studies have demonstrated that PD-L1 on EVs can influence the function of immune cells (Fig. 1)54,77,78. The immunosuppressive
mechanisms of PD-L1-EVs appear to mirror those of membrane-bound PD-L1. They primarily inhibit CD8+ T-cell proliferation, downregulate the production and release of effector molecules, and
promote T-cell apoptosis79. They also inhibit the proliferation of CD4+ T cells while promoting their differentiation into regulatory T cells80,81. The main effect of tumor-associated
macrophages is to promote their differentiation toward the M2 tumor-promoting phenotype82. Furthermore, PD-L1-EVs from head and neck squamous cell carcinoma cells suppress B-cell
proliferation and activity and increase PD-1 and LAG3 expression on their surface; PD-L1-EVs from gastric cancer cells promote myeloid-derived suppressor cell expansion and differentiation,
suggesting that a wide range of immune cells can be affected by PD-L1-EVs83,84. Additionally, PD-L1-EVs perform unique functions. The diffusive nature of EVs in the TME allows PD-L1 to be
not only expressed by one cell but also transferred to other cells. Mauro et al. reported that administering exogenous PD-L1-EVs could rescue the growth of tumors that are incapable of
independently secreting exosomes. Moreover, in prostate cancer, tumors with low PD-L1 expression actively take up PD-L1-EVs from high PD-L1-expressing tumor cells, thereby enhancing overall
antitumor immunity85,86. PD-L1-EVs from head and neck squamous cell carcinoma establish a positive feedback loop between M2 macrophages and Treg cells in the TME, further suppressing CD8+
T-cell proliferation56. PD-L1-EVs also contribute to the establishment of metastatic niches; for example, M2 macrophages in ovarian cancer appear in the peritoneal cavity even before tumor
cells arrive, where they target carnitine palmitoyl-transferase 1 A expression in T cells within the peritoneal cavity through the PD-L1-EVs they release. This enhances fatty acid oxidation
in T cells, increases the levels of intracellular reactive oxygen species, and subsequently damages T cells87. PD-L1-EVs play a critical role in resistance to ICB therapy. High levels of
PD-L1-EVs in patients with cancer suppress the proliferation and activation of T cells, with some T cells exhibiting irreversible functional exhaustion, inherently limiting the effectiveness
of anti-PD-L1 treatments88. Though T cells rescued by anti-PD-L1 therapy can continue to exert antitumor effects, the IFN-γ that is secreted by activated T cells further promotes the
transcription of PD-L1. Additionally, the stimulatory effect of activated T cells on tumor cells prompts the production of more EVs, thereby increasing the levels of PD-L1-EVs and forming a
mechanism that counteracts the efficacy of anti-PD-L1 therapy89. The ability to release PD-L1-EVs varies across different tumor types and states, potentially contributing to the
heterogeneous efficacy of anti-PD-L1 treatments90. Interestingly, researchers reported that inhibiting Rab27a to downregulate EV production greatly increased the efficiency of binding
between PD-L1 on the tumor cell surface and anti-PD-L1 antibodies. In vivo experiments demonstrated a dose-dependent reduction in the quantity of PD-L1 on the tumor surface that was bound to
anti-PD-L1 antibodies by PD-L1-EVs, suggesting that PD-L1 on EVs has greater affinity for anti-PD-L1 antibodies than for PD-L1 on the cell surface91. A previous study92 revealed that tumor
cells can produce abnormally spliced PD-L1 that lacks the transmembrane domain, which is secreted extracellularly and displays high affinity for anti-PD-L1 antibodies, indicating that
tumor-derived PD-L1 is not constant and may undergo changes during packing into EVs or at certain biological stages; this finding warrants further research on the increased affinity of PD-L1
for anti-PD-L1 antibodies. Moreover, the specificity of adhesion molecules on PD-L1-EV surfaces, especially the high expression of cadherins and ICAM1 in these EVs and their tendency to
disperse into the circulation, suggest that circulating PD-L1-EVs could constitute the first line of defense that weakens the efficacy of anti-PD-L1 antibodies. Indeed, the levels of
PD-L1-EVs in the plasma of patients with melanoma were found to be negatively correlated with responsiveness to anti-PD-L1 antibody therapy93. Recently, combining ICB with other radiotherapy
and chemotherapy modalities has emerged as a new treatment approach, but PD-L1-EVs have also been found to contribute to tolerance to these therapies. For example, head and neck squamous
cell carcinoma lines presented an increase in PD-L1-EVs after fractionated radiotherapy or cisplatin treatment, along with a reduction in apoptosis94. The number of glioblastoma stem
cell-derived PD-L1-EVs increased after treatment with temozolomide, increasing intracellular PD-L1 levels after ingestion by tumor cells and subsequently inducing AMPK/ULK1 pathway-mediated
protective autophagy, resulting in tolerance to temozolomide by clearing damaged organelles within cells95. CROSSTALK BETWEEN EVS AND CTLA4 CTLA4 (CD152) is a type I transmembrane
glycoprotein that is homologous to the immunoglobulin CD28. Unlike PD-L1, which functions primarily through EVs, CTLA4 exerts its immunosuppressive effects by continuous flipping between the
inside and outside of the cell membrane to bind to its ligands. Owing to efficient endocytosis, CTLA4 accumulates in the cytoplasm. Upon the production of costimulatory signals generated by
the binding of CD28 with its ligands CD80/CD86 and the TCR with MHC, CTLA4 is transported to the cell surface, where it competes with CD28 to bind to CD80/CD86, thereby mediating T-cell
nonresponsive conditions and recruiting SHP2 to activate T-cell inhibitory signals96. CTLA4 is predominantly expressed on the surface of regulatory T cells and activated CD8+ T cells, and
EVs in the tumor microenvironment further upregulate its expression to create an inhibitory milieu3,97. EVs derived from lymphomas increase CTLA4 expression on CD8+ T cells, converting them
into regulatory CD8+ T cells that perform immunosuppressive functions and secrete the inhibitory cytokines IL10 and TGF-β98. However, it is unclear whether this upregulation occurs through
increased transcription and translation of CTLA4 or by promoting its presentation on the cell surface. EVs carrying lymphoma surface antigens can preferentially bind to chimeric antigen
receptor-modified T cells that are used in therapy, increasing CTLA4 expression on their surface and impairing their tumor-killing ability99. It appears that ICPs can also interfere with
each other through EVs. PD-L1-EVs from esophageal squamous cell carcinoma decrease the ratio of circulating follicular helper T cells to follicular regulatory T cells and upregulate CTLA4 in
the former, inhibiting follicular helper T cell differentiation by preventing the binding of CD28 to its ligands, leading to high expression of ICOS and activation of downstream
PI3K100,101. As more studies have shown that CTLA4 is distributed on other cell surfaces, the related roles of EVs are expanding102 and EVs derived from hepatic stellate cells carrying
circWDR25 enter other stellate cells, sponge miR-4474-3p, and upregulate ALOX15, thereby promoting CTLA4 expression103. In recent years, studies have shown that CTLA-4 is loaded into EVs,
where it plays a role in suppressing T-cell function and promoting T-cell apoptosis104,105. EVs derived from triple-negative breast cancer cells carry CTLA-4, which can induce irreversible
apoptosis in CD8+ T cells. However, the use of anti-CTLA-4 antibodies does not significantly mitigate this effect, suggesting that the apoptotic effect of CTLA-4-EVs is likely mediated by
the stress-induced apoptosis of T cells triggered by the influx of extracellular components, including CTLA-4104. Furthermore, EVs from tumor cells of patients with cachexia and
hepatocellular carcinoma carrying CTLA-4 have been shown to promote tumor cell proliferation and metastasis through the PTEN/CD44 pathway within the tumor microenvironment106. These findings
indicate that the functionality of this membrane-bound receptor is further expanded upon its incorporation into EVs. CROSSTALK BETWEEN EVS AND TIM3 TIM3 is a member of the T-cell
immunoglobulin and mucin domain (TIM) gene family and is classified as a type I transmembrane protein. It is composed of an N-terminal immunoglobulin variable (V) domain and five atypical
cysteines, a mucin stalk, a transmembrane domain, and a cytoplasmic tail3,107. Its immunosuppressive function relies on its interactions with multiple ligands, including C-type lectin
galectin-9 (Gal-9), high mobility group box 1 (HMGB1), carcinoembryonic antigen-related cell adhesion molecule 1 (CEACAM1), and the nonprotein ligand phosphatidylserine (PS)108. Each of
these ligands has a specific mechanism for binding to TIM3, leading primarily to the phosphorylation of its cysteine residues. Following ligand binding, HLA-B-associated transcript 3 (BAT3)
disassociates from the cytoplasmic tail, allowing the tyrosine kinase FYN to bind and generate T-cell inhibitory signals. However, extensive researches have confirmed that different ligands
can induce unique effects upon binding to TIM3 under various conditions3,109. TIM3 is expressed on a variety of cell surfaces and is found predominantly on T cells, NK cells, macrophages,
dendritic cells, and mast cells107. It is highly expressed on NK cells but functions mainly in T-cell suppression, is localized on T-cell membrane rafts and is recruited to immunological
synapses upon T-cell activation to exert its inhibitory effect110,111. Recent studies have shifted this perspective, showing that TIM3 expression on various myeloid-derived cells appears to
be greater than that on T cells112, contributing to a systemic immunosuppressive environment through mechanisms such as promoting M2 macrophage differentiation and inhibiting DNA recognition
receptors in antigen-presenting cells113,114. The interaction between TIM3 and EVs is notably complex, with several ligands found to be transported via EVs. EVs derived from nasopharyngeal
carcinoma cells carrying Gal-9 bind to TIM3 on dendritic cells (DCs), transforming them into regulatory DCs. This interaction downregulates the release of costimulatory molecules and
inflammatory factors while concurrently increasing the expression of PD-1 and CTLA4 on their surface. Additionally, Gal-9-carrying EVs inhibit the migration of DCs, thereby impairing their
ability to efficiently enter the tumor microenvironment and exert their functions112. A recent study has suggested that the binding of Gal-9 to TIM3 on the surface of DCs does not directly
activate downstream inhibitory signals. Instead, its dimerization domain promotes the aggregation of TIM3 on the DC surface, enhancing the binding efficiency of the HMGB1-antigen DNA complex
to TIM3. This, in turn, competitively inhibits the binding of HMGB1 to endocytic receptors, thereby preventing the entry of tumorigenic DNA into DCs and the induction of the
antigen-presenting response113. However, research on this EV-mediated mechanism is still lacking. Given the long-distance, multisite transport capabilities of EVs, TIM3-related EVs could
regulate tumor immune evasion beyond the TME115. In pleural effusions from patients with lung cancer, EVs not only express prometastatic angiogenic proteins but also exhibit high surface
expression of CEACAM1. These EVs bind to TIM3 on the surface of CD3+CD4-CD8- double-negative T (DNT) cells in the effusion, diminishing their cytotoxic effect on free tumor cells within the
malignant effusion and thus fostering a conducive environment for tumor metastasis115,116. TIM3 itself can also be transferred between cells via EVs, a phenomenon observed in osteosarcoma
and melanoma. TIM3-EVs suppress the function of CD4+ T cells and induce the differentiation of M2-type macrophages. Unlike CTLA4-EVs, EVs carrying TIM3 seem to perform functions similar to
those of its membrane-bound form117,118. Although the functional mechanisms of TIM3-EVs remain unclear, it is hypothesized that they primarily increase the expression of TIM3 within target
cells after entry, but this requires further experimental validation. In summary, considering the numerous ligands and complex modes of action associated with TIM3, its exploration presents
a compelling area of research. CROSSTALK BETWEEN EVS AND LAG3 LAG3 (CD223) plays a pivotal role in limiting T-cell activation, and inhibitors that target LAG3 represent the third ICB
therapeutic protocol approved by the FDA. When used in combination with anti-PD-1 antibodies for the treatment of patients with melanoma, these agents have demonstrated substantial efficacy
and safety, with significant clinical potential119. LAG3, an inhibitory receptor belonging to the type 1 Ig superfamily, has a structure similar to that of CD4 but has a greater affinity for
MHC class II molecules108. Upon antigen activation of the TCR/CD3 complex, binding with LAG3 is mediated through a KIEELE motif, a glutamic acid‒proline dipeptide repeat (EP motif), and a
serine phosphorylation site (S484) in the cytoplasmic domain of LAG3, which mediates downstream T-cell inhibitory signals or inhibits TCR signal transduction by disrupting the association of
the CD4/CD8 coreceptor with cytoplasmic tyrosine kinases3,120. Research on the interaction between LAG3 and EVs is limited, but a substantial body of evidence confirms that
immunosuppressive EVs can increase the expression of LAG3 on T-cell surfaces, indicating that this effect is dependent on the miRNAs carried by EVs, which increase LAG3 transcription87,121.
Posttranscriptional regulation of LAG3 has been found to be associated with N6-methyladenosine (m6A), particularly through the balance between the RNA-binding enzyme YTHDF1, which recognizes
m6A sites on LAG3 mRNA and assists in translation, and the RNA demethylase ALKBH5122. A recent study on M2 macrophage-derived EVs has indicated that they carry miR-21-5p, which inhibits the
expression of the m6A methyltransferase METTL3, thereby limiting mRNA methylation. Although direct evidence of the influence of METTL3 on LAG3 expression is lacking, the regulatory effect
of EVs on m6A could also impact LAG3 expression123. Tumor endothelial cell-derived EVs that enter circulation can induce high expression of the exhaustion marker LAG3 on T cells at distant
sites, creating an immunosuppressive environment that is favorable for tumor metastasis; this shows how EVs can assist in establishing systemic immunosuppression associated with LAG397.
Other ligands for LAG3 have been identified, including galectin-3 (Gal-3), liver and lymph node sinusoidal endothelial cell c-type lectin (LSECtin), fibrinogen-like protein 1 (FGL1), and
preformed fibrils of α-synuclein (α-syn PFF); however, the roles of these ligands within the functional spectrum of LAG3 remain controversial120. For example, FGL1 has been found to interact
specifically and physiologically with LAG3 with high affinity and can mediate LAG3’s T-cell inhibitory function independently of MHC class II, although this conclusion is disputed across
different studies124,125. However, FGL1 levels are widely believed to be associated with poor patient prognosis, especially as recent study has shown that FGL1 levels in circulating EVs from
patients with lung adenocarcinoma more sensitively reflect tumor progression and correlate with responsiveness to anti-PD-1 therapy than free FGL1 in plasma126, suggesting a complementary
immunosuppressive function of EV-derived FGL1 to PD-L1. Additionally, an increasing number of studies on the role of Gal-3 in mediating T-cell functional suppression via LAG3 have been
published127,128, with substantial amounts of Gal-3-expressing EVs being extracted from head and neck squamous cell carcinoma cell lines, indicating that Gal-3 may interact with LAG3 through
EVs129. CROSSTALK BETWEEN EVS AND ICOS ICOS is an immune checkpoint expressed specifically on the surfaces of activated CD4+ and CD8+ T cells. Owing to its structural similarity to CD28 and
CTLA4, as well as its ligand B7-H2, which belongs to the B7 protein family alongside PD-L1, ICOS is also referred to as an alternative immune checkpoint130. ICB drugs targeting ICOS are in
development, but most results indicate that their efficacy is primarily synergistic with those of other ICB medications131,132. ICOS mediates tumor progression through T-cell regulation, but
this regulatory effect is considered bidirectional; on the one hand, ICOS on Treg cells induces the production of IL-10, mediating immune suppression; on the other hand, ICOS on CD4+ and
CD8+ T cells mediates the production of effector molecules such as IFN-γ and TNFα133. Interestingly, adipocyte-derived EVs from obese patients with lung adenocarcinoma carrying miR-27a-3p
can directly inhibit ICOS transcription, subsequently reducing the level of IFN-γ released by T cells134. Moreover, when circulating Tfh cells and regulatory T (Tfr) cells are cocultured
with PD-L1-EVs from esophageal squamous cell carcinoma, ICOS expression increases only on Tfr cells, consistent with the distinct functions of ICOS on these cells101. ICOS engagement on Tfh
cells can mediate B-cell immune-promoting functions, whereas ICOS engagement on Tfr cells, which is dependent on ICOS for differentiation, inhibits normal B-cell functions upon binding,
highlighting the complex nature of ICOS function owing to its opposite effects on different cell types135,136. Additionally, ICOS can move between cells via EVs, with EVs released from CD4+
T cells selectively enriched with ICOS and CD40 on their surface assisting in the physiological interaction of CD40 with its ligand as an immunostimulant137. According to a previous clinical
study138, patients with persistently high surface expression of ICOS on peripheral blood CD4+ T cells who receive CTLA4 blockade therapy exhibited greater clinical benefits. However, a
recent study revealed that the levels of ICOS in peripheral blood EVs are unrelated to the response and prognosis of patients with gastric cancer receiving ICB therapy139. CROSSTALK BETWEEN
EVS AND OTHER ICPS The functionality of ICPs is not isolated; in fact, the inhibition of one ICP often leads to compensatory upregulation of other ICPs, posing a significant challenge in
current ICB therapy140. Exploring novel ICPs is increasingly critical in advancing ICB therapy, and some novel ICPs have been shown to be associated with EVs, suggesting the important role
of ICP-related EVs in tumor immunity141. B7-H3 (CD276), a member of the B7 family similar to PD-L1 and part of the B7-CD28 interaction, aids in tumor immune evasion and metastasis142.
Similar to PD-L1, B7-H3 promotes the production of EVs. In medulloblastoma cells, high B7-H3 expression upregulates PIK3C2 (a class II PI3K enzyme), which is related to vesicle formation143.
In addition, high B7-H3 expression in EVs is correlated with a decreased immune response and adverse patient outcomes143. B7-H3 derived from colorectal cancer cell-derived EVs can be taken
up by vascular endothelial cells, where it then upregulates and activates the AKT1/mTOR/VEGFA pathway. This process promotes endothelial cell migration and tubule formation, resulting in a
different function from that of B7-H3 on the cell surface144. Notably, B7-H3 is expressed primarily on antigen-presenting cells, but it is unclear whether EVs from these cells also carry
B7-H3 and what their functions are145. T-cell immunoreceptor with Ig and immunoreceptor tyrosine-based inhibitory motif (TIGIT) is another kind of coinhibitory receptor that forms an
antagonistic network with the costimulatory receptors CD226 (DNAM-1), CD96, and CD112R146. As a direct target of Foxp3, TIGIT is considered a Treg marker influenced by immunosuppressive EVs
in the tumor microenvironment, indicating active Treg cells99,147. Indoleamine 2,3-dioxygenase (IDO), another endogenous immune checkpoint, catalyzes the breakdown of tryptophan to
kynurenine (L-kyn), inhibiting effector T-cell activation and promoting Foxp3+ Treg differentiation through kynurenine production148. IDO can be transferred through EVs, which is correlated
with poor outcomes in patients with tumors149. In glioblastoma, high IFN-γ expression induces PD-L1 and IDO expression and increases their levels on EVs. However, IDO-EVs do not directly
inhibit T-cell function; instead, they induce the differentiation of nonclassical monocytes, thereby indirectly downregulating T-cell proliferation150. Furthermore, L-kynurenine can also be
loaded into EVs. When these EVs are taken up by endothelial cells, L-kynurenine increases intracellular NAD+ levels and Sirt3 acetylation, enhancing mitochondrial function and promoting
endothelial cell proliferation, thus inducing tumor angiogenesis151. These interactions with EVs expand the functional spectrum of ICPs. CLINICAL PROSPECTS OF ICP-RELATED EVS EVS AS
PREDICTORS OF ICB TREATMENT Clinical research on ICB therapies has undergone significant advancements in recent years, with the FDA recently approving five drugs that target PD-1 or PD-L1
(nivolumab, pembrolizumab, atezolizumab, durvalumab, and avelumab) for inclusion in treatment guidelines for 11 types of cancer6. Drugs that target newly identified ICPs, such as relatlimab
and fianlimab that target LAG3, are also being quickly applied in clinic120,152. Early-phase clinical trials for ICB drugs that target TIM3 are underway (NCT03680508, NCT05216835). Despite
the rapid development of ICB therapies, challenges such as heterogeneous patient responses and common adverse reactions limit the clinical adoption of ICB drugs. Discussions about the safety
and efficacy of the earliest FDA-approved CTLA4-blocking drugs, ipilimumab and tremelimumab, continue to this day153,154. Although that combination of different ICB drugs has improved
therapeutic outcomes, recent reports have highlighted an increased incidence of immune-related adverse events, such as hypophysitis or hypopituitarism154,155. Therefore, there is a critical
need for biomarkers that can monitor the effects of ICB therapy and predict related adverse reactions. In a pancancer clinical study, researchers reported that PD-L1 levels in tumor tissues
can reflect patient responsiveness to ICB therapy156, suggesting that ICP expression levels may predict patient benefit from ICB therapy. However, this study relied primarily on genomic
analysis and immunohistochemistry of tumor tissues to assess PD-L1 levels. Because most ICPs function as membrane-bound entities and are concentrated in specific locations rather than in
circulation, it is difficult to monitor ICP levels via less invasive sample collection methods. EVs, however, serve as an exception; they have the membrane structure that is necessary for
ICP attachment and can move within the circulation, offering inherent advantages in monitoring circulating ICP levels. Indeed, liquid biopsies to collect ICP-carrying EVs have been found in
multiple studies to increase cancer detection rates and predict patient prognosis157,158,159. Furthermore, researches indicate that the expression of ICPs on the vesicle surface is
influenced by disease duration and treatment measures160,161,162. Compared with soluble ICPs, the more stable and accessible carrier form of ICPs on EVs presents significant potential as a
biomarker (Table 2). Single-molecule array technology has been used for the high-sensitivity detection of protein biomarkers in plasma EVs that are isolated from patients with B lymphoma,
and the results revealed that PD-L1-EV levels are correlated with poor patient prognosis and response to chemotherapy, increasing interest in monitoring PD-L1-EVs to assess responses to
immune checkpoint therapy163. The phenomenon of detecting ICB efficacy through circulating EVs was first identified in melanoma patients treated with pembrolizumab, where plasma PD-L1-EV
levels were positively correlated with intracellular Ki-67 levels in CD8+ T cells. Moreover, the fold increases in total circulating PD-L1, microvesicle PD-L1, and PD-L1, which are excluded
by EVs, were lower in distinguishing responders from PD-L1-EVs89. Traditional EV analysis methods rely primarily on ultracentrifugation for EV separation followed by Western blotting and
ELISA for protein analysis, but the low throughput, low efficiency, and high cost of these methods greatly limit their clinical application164. Specifically, the clinical monitoring EVs
carrying specific ICPs requires more high-purity, low-cost EV separation methods. Current mainstream EV separation techniques include size-based separation, ultracentrifugation,
immunoaffinity capture, precipitation methods, microfluidics-based isolation techniques, filtration, or their combinations165. Among these, immunoaffinity capture is particularly favored for
clinical research because of its direct specificity for distinguishing EVs expressing or carrying ICPs. Although antibody-based immunoaffinity capture methods may occupy antigenic sites on
EV surfaces, affecting further analysis, and the risk of nonspecific antibody binding cannot be ignored, it remains a viable option for quantifying PD-L1-EVs. For example, anti-PD-L1
antibody-conjugated gold nanorods attached to EVs produce varying scattering intensities in localized surface plasmon resonance (LSPR)-based nanoplasmonic biosensors based on the expression
levels of PD-L1 on each EV; this approach enabled not only semiquantification of total PD-L1-EVs in samples but also differentiation of PD-L1 content on individual EV166. The active
development of amplifiers for LSPR PD-L1-EV signals is also underway167. However, the detection of PD-L1-EVs in this manner provides information about only the PD-L1 levels of EVs from all
cellular sources in patients, including a significant proportion of physiologically released PD-L1-EVs; this limitation reduces the accuracy of this assessment. Researchers have designed a
process specifically for patients with melanoma that relies on aptamers to recognize tumor cell-derived PD-L1-EVs. Aptamers are short nucleotide ligands that are smaller than conventional
antibodies, allowing easier engineering. Additionally, glycosylated PD-L1, which may be less readily identified by antibodies, can still be recognized by aptamers168. The designed aptamers
bind to PD-L1 on the surface of EVs and the tumor marker EpCAM. Subsequently, aptamers that bind to different proteins on the same membrane are linked, and with the assistance of a proximity
ligation assay, tumor cell-derived PD-L1-EVs can be quantified using droplet digital PCR. This process has been shown to provide greater accuracy in patients with melanoma and may have
better potential for predicting responses to ICB therapy169. To further reduce the sample volume requirement for quantitative detection with aptamers, another group of researchers combined
two types of aptamer probes, one that binds to PD-L1 on the surface of EVs and one that binds to EV-derived miR-21 in EV lysis fluid, using gold nanorods. The addition of two fuel strands
mediates probe binding and release, allowing a dual probe to repeatedly bind and release fluorescence groups with PD-L1-EVs and miR-21, thus amplifying the signal for biomarker detection170.
A novel approach involves attaching the PD-L1 aptamer to ternary metal-metalloid palladium-copper-boron alloy microporous nanospheres that can conduct electrical signals, allowing the
detection of PD-L1-EV levels based on characteristic electrical signals and further enhancing the sensitivity of diagnosis in patients with non-small cell lung cancer171. The aforementioned
immunological affinity capture strategy still relies primarily on ultracentrifugation for the initial EV purification step. Although this method is considered the gold standard for purifying
EVs in current clinical practice, its complexity and high cost limit further dissemination165. Magnetic beads with immunological affinity represent a potential alternative, allowing EVs to
be captured via their direct adhesion to EVs. Researchers have designed TiO2 magnetic nanoparticles that bind indiscriminately to the hydrophilic phosphate heads of exosomal phospholipids,
followed by the addition of the PD-L1 marker “Au@Ag@MBA”, which is required for surface-enhanced Raman scattering (SERS) immunoassays; this approach allows the precise quantification of
PD-L1-EVs from only 4 μl of a plasma sample172. A recent study173 introduced artificial Hoogsteen hydrogen bonding interactions to form triple-helix molecular probes (THMPs), which produce a
fluorescent signal upon binding with PD-L1. However, magnetic bead-based separation strategies also have considerable limitations, such as high costs and poor reproducibility, necessitating
further optimization. Interestingly, one study explored the relationship between PD-L1-EVs derived from the gut microbiome and the response of patients with melanoma to ICB therapy,
yielding positive results174. This finding highlights the diverse sources of EVs that carry PD-L1, which has great potential to meet various clinical needs. Additionally, the levels of
circulating PD-L1-EVs in patients with early osteosarcoma were found to be correlated with long-term prognosis, offering a new perspective for monitoring this cancer type, which lacks
effective prognostic markers175. The exploration of the potential of other ICPs as biomarkers is currently in a very early stage. However, some studies have indicated that the levels of
CTLA-4, LAG-3, and TIM-3 originating from cells in patient tumor tissues are associated with survival times post-ICB treatment176,177. Furthermore, high levels of TIM3-EVs and Gal-9-EVs in
the plasma of patients with non-small cell lung cancer are clearly correlated with poor prognosis, suggesting a promising start178. Notably, high levels of ubiquitin-like with PHD and ring
finger domain 1 RNA within the plasma EVs of patients with hepatocellular carcinoma are associated with a low response to anti-PD-1 therapy, as it degrades miR-449c-5p, thereby upregulating
TIM-3 expression in NK cells179. The observation of high levels of PD-1-EVs in patients who are resistant to ICB therapy underscores the clinical significance of this research area82. The
level of FGL1, the ligand for LAG-3, in plasma EVs are related to low responsiveness to PD-1 therapy, a phenomenon not observed when total FGL1 levels are measured126. This correlation
between EV contents and ICP levels further expands the pool of potential biomarkers for predicting ICB therapy response. THE ROLE OF EVS IN INCREASING ICB TREATMENT EFFICACY Monoclonal
anti-ICP antibodies remain the most common clinical treatment to date. However, their instability has prompted researchers to explore other potential ICB alternatives. In particular, given
the close relationship between EVs and ICPs, the exploration of ICB alternatives from the perspective of EVs is increasingly gaining attention from researchers (Fig. 2). As we summarized in
our previous article12, EVs have a bidirectional effect on tumor progression due to the diversity of their cargo, a phenomenon that also occurs in the interaction between EVs and ICPs. In
colorectal cancer, EVs derived from adipose mesenchymal stem cells carry miR-15a, which targets lysine demethylase 4B (KDM4B) and inhibits PD-L1 expression by downregulating the binding of
homeobox C4 (HOXC4) to the PD-L1 promoter180. The expression of miR-16-5p derived from the serum EVs of patients with lung adenocarcinoma who were treated with anti-PD-L1 antibodies
increased and could further inhibit PD-L1 expression181. The artificial administration of melatonin elevated the levels of several miRNAs in gastric cancer cell-derived EVs that inhibit
macrophage PD-L1 expression22. Additionally, EV cargo influences the transcription of PD-L1 through the PI3K/Akt and STAT1/3 pathways. Conversely, downregulating these pathways significantly
inhibits ICPs on the cell and EV surfaces29,182. Designing inhibitors that target miRNAs carried by EVs that regulate PD-L1 has also shown promising results in both cell and animal
experiments. Although excessive accumulation of ROS inhibits M2 macrophage uptake of PD-L1-inhibitory EVs, neutralization of ROS with N-acetyl-L-cysteine (NAC) increases the levels of
miR-155-5p in tumor-derived EVs that are taken up by macrophages43. Interestingly, when cells cannot endure endoplasmic reticulum stress, immunogenic cell death is initiated in tumor cells,
and they release tumor antigens and damage-associated molecular pattern (DAMP) to activate surrounding immune cells. When cells were with bafilomycin A1 (bafA1) and EVs carrying misfolded
proteins were collected, the induction of endoplasmic reticulum stress in tumor cells after EV uptake caused tumor cell death and induced T-cell activation, significantly enhancing the
therapeutic effect when combined with anti-PD-1 treatment183. Direct modulation of the production and release processes of PD-L1-carrying EVs has also shown promise for clinical application
(Fig. 2). For example, the use of sulfisoxazole to downregulate Rab27a expression led to a decrease in PD-L1 levels in EVs182. GW4869, which blocks EV secretion by inhibiting nSMase
synthesis, synergizes with anti-PD-L1 therapy in 4T1 breast cancer-bearing mice58. Endothelin receptor A (ETA) is a member of the GPCR family and is extensively involved in the regulation of
proteins related to MVB maturation, the fusion of MVBs with the plasma membrane, and autophagic lysosomal degradation184. Macitentan, an antimicrobial drug that targets ETA, can also
decrease the ability of tumor cells to produce PD-L1-EVs. In addition, it inhibits the capacity of PD-L1 on the surface of EVs to bind with PD-1 on target cells185. Additionally, directly
disrupting the functionality of the Golgi apparatus in tumor cells has shown to potently suppress the production of PD-L1-EVs186. Several pharmacologically potent substances, including
tipifarnib, neticonazole, climbazole, ketoconazole, triademenol, manumycin A, and nexinhibs, have shown to inhibit exosome production by reducing the expression of proteins that are
essential for ESCRT-dependent exosome formation and the Rab27a transport mechanism55,88. Moreover, inhibitors of exosomes that specifically target the ESCRT-independent enzyme
N-sphingomyelinase (nSMase), such as GW4869 and spiroepoxide, can block the release of exosomes187,188. Despite the current lack of research connecting these drugs with PD-L1-related
therapies, this direction has considerable potential. Owing to the nonimmunogenicity, nontoxicity, and degradation resistance of EVs, they are widely used as drug delivery carriers (Fig. 2).
The binding of siRNAs to target genes induces sequence-specific degradation, and the protocol of loading siRNAs into EVs through electroporation and introducing them into organisms has
shown high efficiency and safety. Exosomes loaded with PD-L1 siRNA or CTLA-4 siRNA precisely reduce the expression of PD-L1 or CTLA-4 in CRC cells23. Loading CD38 siRNA into EVs derived from
bone marrow mesenchymal stem cells, which are then taken up by liver cancer cells, downregulates CD38 enzyme activity and adenosine secretion, thereby promoting the differentiation of
macrophages toward the M1 phenotype and reducing the release of PD-L1-EVs189. Additionally, researchers have extracted EVs carrying large amounts of target proteins from plasmids to assist
in ICB therapy. For example, EVs from epithelial cells carry a large amount of OX40L, which, by activating the function of CD4+ T cells, further enhances the therapeutic effect against
CTLA-424. Recent research has revealed that increasing the activity of tumor-specific immune cells within the human body can significantly increase the efficacy of ICB therapy190. The
interaction between these cells and EVs has been found to further amplify this effect. Dendritic cells (DCs) that are educated by tumor-derived EVs promote the proliferation and function of
tumor-specific T cells. Moreover, these cells can release EVs carrying tumor-specific antigens, which, when ingested by tumor cells, increase the immunogenicity of tumor cells. When these
EVs bind to T cells, they can promote T-cell activation and function. Although the function of T cells is eventually exhausted by PD-L1 in the tumor microenvironment, the significantly
increased baseline number of T cells results in a significant increase in the number of T cells that regain activity after anti-PD-L1 treatment191,192. When reinfused into mice,
tumor-specific neoantigen peptides that are carried by serum EVs from mice are efficiently taken upby dendritic cells, in turn inducing the production of more specific T cells and further
facilitating anti-PD-1 therapy193. Additionally, because tumor-activated dendritic cells migrate to lymph nodes and dendritic cell-derived EVs are able to home to their parent cells,
subcutaneous injections of dendritic cell-derived EVs carrying tumor antigens rapidly accumulate in tumor-draining lymph nodes194. By using lipid anchoring technology to modify anti-CTLA4
antibodies on the surface of DC-derived EVs, these EVs can efficiently bind to receptors on the surface of CD4+ T cells in lymph nodes. These EVs rely on antigens, MHC molecules, and CD80 on
their surface to activate T cells while also blocking the transmission of T-cell inhibitory signals195. LIMITATIONS IN ICP-RELATED EV RESEARCH Despite substantial advances in the research
of EVs and ICPs over the past few decades, studies that consider them as functionally interacting factors remain insufficient. The complex network of EV‒ICP interactions remains a field with
many unanswered questions, especially for newly discovered ICPs such as CTLA4, TIM3, LAG3, and ICOS. Although the roles of ICPs in intercellular regulatory processes have been partially
elucidated, their presence in EVs has not received the same level of attention as that of PD-L1. Given the diversity of EV sources and their unique mechanisms of action, it is plausible that
ICPs associated with EVs may perform distinct and unexpected functions. Further investigations into these various ICPs in EVs are warranted and may significantly expand the horizons of
tumor immunotherapy. With advances in EV isolation and identification technologies, the theoretical feasibility of using ICPs as biomarkers for cancer diagnosis and prognosis is increasing.
The diversity and programmability of EV cargo also endow them with potential as mediators for ICB therapy. However, these diagnostic and monitoring methods lack long-term observational
results, and their efficacy in practical situations remains uncertain. Additionally, most researches on the reprogramming of EVs for tumor treatment are limited to cellular or animal models;
studies on the use of this therapy within the complex human tumor microenvironment seem premature. Another technical bottleneck of such engineered EVs is the lack of methods to efficiently
and consistently obtain EVs that meet the requirements of clinical practice. Nevertheless, researchers have never ceased their efforts to translate EVs into clinical applications. For
example, a recent study discussed the trade-offs involved in developing therapeutic methods using mesenchymal stem cell-derived EVs196. CONCLUSION In this review, we explore the complex
interactions between EVs and key ICPs, including PD-L1, CTLA4, TIM3, LAG3, and ICOS. EVs play a crucial role in the lifecycle of these ICPs, influencing their production, transport, and
functionality. EVs not only carry cargo that can modulate ICP expression but also facilitate the transfer of ICP ligands and receptors between cells, inducing complex regulatory mechanisms
and altering the biological properties of EVs to further tumor progression. This interplay presents a sophisticated functional landscape of ICPs and opens new avenues for their clinical
application. DATA AVAILABILITY Data sharing is not applicable to this article, as no datasets were generated or analyzed during the current study. CHANGE HISTORY * _ 17 JANUARY 2025 The
original online version of this article was revised: In the Funding section of this article the grant number relating to the National Natural Science Foundation of China was incorrectly
given as 82173245, 82300881, 82473062 and should have been 82473062, 82173245, 82300881. _ * _ 20 JANUARY 2025 A Correction to this paper has been published:
https://doi.org/10.1038/s12276-025-01394-4 _ REFERENCES * Linsley, P. S. et al. CTLA-4 is a second receptor for the B cell activation antigen B7. _J. Exp. Med._ 174, 561–569 (1991). Article
CAS PubMed Google Scholar * Xing, C. et al. The roles of exosomal immune checkpoint proteins in tumors. _Mil. Med. Res._ 8, 56 (2021). CAS PubMed PubMed Central Google Scholar *
Gaikwad, S., Agrawal, M. Y., Kaushik, I., Ramachandran, S. & Srivastava, S. K. Immune checkpoint proteins: Signaling mechanisms and molecular interactions in cancer immunotherapy.
_Semin. Cancer Biol._ 86, 137–150 (2022). Article CAS PubMed Google Scholar * Weber, J. Immune checkpoint proteins: a new therapeutic paradigm for cancer-preclinical background: CTLA-4
and PD-1 blockade. _Semin. Oncol._ 37, 430–439 (2010). Article CAS PubMed Google Scholar * He, X. & Xu, C. Immune checkpoint signaling and cancer immunotherapy. _Cell Res._ 30,
660–669 (2020). Article PubMed PubMed Central Google Scholar * Ribas, A. & Wolchok, J. D. Cancer immunotherapy using checkpoint blockade. _Sci. (N. Y., NY)_ 359, 1350–1355 (2018).
Article CAS Google Scholar * Hodi, F. S. et al. Improved survival with ipilimumab in patients with metastatic melanoma. _N. Engl. J. Med._ 363, 711–723 (2010). Article CAS PubMed
PubMed Central Google Scholar * Beaver, J. A. et al. Patients with melanoma treated with an anti-PD-1 antibody beyond RECIST progression: a US Food and Drug Administration pooled analysis.
_Lancet Oncol._ 19, 229–239 (2018). Article CAS PubMed PubMed Central Google Scholar * Heidegger, S. et al. Targeting nucleic acid sensors in tumor cells to reprogram biogenesis and
RNA cargo of extracellular vesicles for T cell-mediated cancer immunotherapy. _Cell Rep. Med._ 4, 101171 (2023). Article CAS PubMed PubMed Central Google Scholar * Wang, A. et al.
Pyroptosis and the tumor immune microenvironment: A new battlefield in ovarian cancer treatment. Biochimica et biophysica acta Reviews on cancer. 2023:189058. * Fang, J. et al. Exploring the
crosstalk between endothelial cells, immune cells, and immune checkpoints in the tumor microenvironment: new insights and therapeutic implications. _Cell Death Dis._ 14, 586 (2023). Article
PubMed PubMed Central Google Scholar * Ye, Z., Chen, W., Li, G., Huang, J., Lei, J. Tissue-derived extracellular vesicles in cancer progression: mechanisms, roles, and potential
applications. _Cancer Metastasis Rev._ (2023). * Théry, C. et al. Minimal information for studies of extracellular vesicles 2018 (MISEV2018): a position statement of the International
Society for Extracellular Vesicles and update of the MISEV2014 guidelines. _J. Extracell. vesicles_ 7, 1535750 (2018). Article PubMed PubMed Central Google Scholar * Guo, S. et al. The
role of extracellular vesicles in circulating tumor cell-mediated distant metastasis. _Mol. Cancer_ 22, 193 (2023). Article CAS PubMed PubMed Central Google Scholar * Yue, M. et al.
Extracellular vesicles remodel tumor environment for cancer immunotherapy. _Mol. Cancer_ 22, 203 (2023). Article CAS PubMed PubMed Central Google Scholar * Alia Moosavian, S., Hashemi,
M., Etemad, L., Daneshmand, S. & Salmasi, Z. Melanoma-derived exosomes: Versatile extracellular vesicles for diagnosis, metastasis, immune modulation, and treatment of melanoma. _Int.
Immunopharmacol._ 113, 109320 (2022). Article CAS PubMed Google Scholar * Fyfe, J., Dye, D., Razak, N. B. A., Metharom, P. & Falasca, M. Immune evasion on the nanoscale: Small
extracellular vesicles in pancreatic ductal adenocarcinoma immunity. _Semin. Cancer Biol._ 96, 36–47 (2023). Article CAS PubMed Google Scholar * Li, L. et al. Exosomes as a modulator of
immune resistance in human cancers. _Cytokine Growth Factor Rev._ 73, 135–149 (2023). Article CAS PubMed Google Scholar * Wang, H. et al. High throughput and noninvasive exosomal PD-L1
detection for accurate immunotherapy response prediction via Tim4-functionalized magnetic core-shell metal-organic frameworks. _Anal. Chem._ 95, 18268–18277 (2023). Article CAS PubMed
Google Scholar * Park, S. J. et al. Clinical significance of serum-derived exosomal PD-L1 expression in patients with advanced pancreatic cancer. _BMC cancer_ 23, 389 (2023). Article CAS
PubMed PubMed Central Google Scholar * Xu, P. et al. Clinical significance of plasma PD-L1(+) exosomes in the management of diffuse large B cell lymphoma. _Ann. Hematol._ 102, 2435–2444
(2023). Article CAS PubMed Google Scholar * Wang, K. et al. Melatonin enhances anti-tumor immunity by targeting macrophages PD-L1 via exosomes derived from gastric cancer cells. _Mol.
Cell. Endocrinol._ 568-569, 111917 (2023). Article CAS PubMed Google Scholar * Li, J. et al. Exosomes-delivered PD-L1 siRNA and CTLA-4 siRNA protect against growth and tumor immune
escape in colorectal cancer. _Genomics_ 115, 110646 (2023). Article CAS PubMed Google Scholar * Kugeratski, F. G. et al. Engineered immunomodulatory extracellular vesicles derived from
epithelial cells acquire capacity for positive and negative T cell co-stimulation in cancer and autoimmunity. bioRxiv : the preprint server for biology. (2023). * Akhtar, M., Rashid, S.
& Al-Bozom, I. A. PD-L1 immunostaining: what pathologists need to know. _Diagnostic Pathol._ 16, 94 (2021). Article CAS Google Scholar * Akinleye, A. & Rasool, Z. Immune
checkpoint inhibitors of PD-L1 as cancer therapeutics. _J. Hematol. Oncol._ 12, 92 (2019). Article PubMed PubMed Central Google Scholar * Chamoto, K., Yaguchi, T., Tajima, M. &
Honjo, T. Insights from a 30-year journey: function, regulation and therapeutic modulation of PD1. _Nat. Rev. Immunol._ 23, 682–695 (2023). Article CAS PubMed Google Scholar * Glaviano,
A. et al. PI3K/AKT/mTOR signaling transduction pathway and targeted therapies in cancer. _Mol. Cancer_ 22, 138 (2023). Article CAS PubMed PubMed Central Google Scholar * Xu, W., Chen,
Y., Zhang, Z., Jiang, Y. & Wang, Z. Exosomal PIK3CB promotes PD-L1 expression and malignant transformation in esophageal squamous cell carcinoma. _Med. Oncol. (Northwood, Lond., Engl.)_
40, 221 (2023). Article CAS Google Scholar * Xu, D. et al. Tumor-derived small extracellular vesicles promote breast cancer progression by upregulating PD-L1 expression in macrophages.
_Cancer cell Int._ 23, 137 (2023). Article CAS PubMed PubMed Central Google Scholar * Zhao, L. et al. LOXL4 shuttled by tumor cells-derived extracellular vesicles promotes immune escape
in hepatocellular carcinoma by activating the STAT1/PD-L1 Axis. _J. Immunother. (Hagerstown, Md : 1997)_ 47, 64–76 (2024). CAS Google Scholar * Han, N., Zhou, D., Ruan, M., Yan, M. &
Zhang, C. Cancer cell-derived extracellular vesicles drive pre-metastatic niche formation of lymph node via IFNGR1/JAK1/STAT1-activated-PD-L1 expression on FRCs in head and neck cancer.
_Oral. Oncol._ 145, 106524 (2023). Article CAS PubMed Google Scholar * Lütge, M., Pikor, N. B. & Ludewig, B. Differentiation and activation of fibroblastic reticular cells.
_Immunological Rev._ 302, 32–46 (2021). Article Google Scholar * Yin, L. & Wang, Y. Extracellular vesicles derived from M2-polarized tumor-associated macrophages promote immune escape
in ovarian cancer through NEAT1/miR-101-3p/ZEB1/PD-L1 axis. _Cancer Immunol., Immunother. : CII_ 72, 743–758 (2023). Article CAS PubMed Google Scholar * Wang, Q. M. et al. Exosomal
lncRNA NEAT1 inhibits NK cell activity to promote multiple myeloma cell immune escape via an EZH2/PBX1 axis. _Mol. Cancer Res_. (2023). * Yang, K., Zhang, J. & Bao, C. Exosomal circEIF3K
from cancer-associated fibroblast promotes colorectal cancer (CRC) progression via miR-214/PD-L1 axis. _BMC Cancer_ 21, 933 (2021). Article CAS PubMed PubMed Central Google Scholar *
Zhao, B. et al. Lnc-CCNH-8 promotes immune escape by up-regulating PD-L1 in hepatocellular carcinoma. _Mol. Ther. Nucleic acids_ 35, 102125 (2024). Article CAS PubMed PubMed Central
Google Scholar * Cubillos-Ruiz, J. R., Bettigole, S. E. & Glimcher, L. H. Tumorigenic and immunosuppressive effects of endoplasmic reticulum stress in cancer. _Cell_ 168, 692–706
(2017). Article CAS PubMed PubMed Central Google Scholar * Fu, X. et al. Endoplasmic reticulum stress, cell death and tumor: association between endoplasmic reticulum stress and the
apoptosis pathway in tumors (Review). _Oncol. Rep._ 45, 801–808 (2021). Article CAS PubMed PubMed Central Google Scholar * Yuan, Y. et al. Endoplasmic reticulum stress promotes the
release of exosomal PD-L1 from head and neck cancer cells and facilitates M2 macrophage polarization. _Cell Commun. Signal._ 20, 12 (2022). Article CAS PubMed PubMed Central Google
Scholar * Yan, D., Wang, H. W., Bowman, R. L. & Joyce, J. A. STAT3 and STAT6 signaling pathways synergize to promote cathepsin secretion from macrophages via IRE1α activation. _Cell
Rep._ 16, 2914–2927 (2016). Article CAS PubMed PubMed Central Google Scholar * Liu, J. et al. Endoplasmic reticulum stress causes liver cancer cells to release exosomal miR-23a-3p and
up-regulate programmed death ligand 1 expression in macrophages. _Hepatol. (Baltim., Md)_ 70, 241–258 (2019). Article CAS Google Scholar * Li, X. et al. Reactive oxygen species reprogram
macrophages to suppress antitumor immune response through the exosomal miR-155-5p/PD-L1 pathway. _J. Exp. Clin. cancer Res._ 41, 41 (2022). Article CAS PubMed PubMed Central Google
Scholar * Yuan, Y. et al. Exosomal O-GlcNAc transferase from esophageal carcinoma stem cell promotes cancer immunosuppression through up-regulation of PD-1 in CD8(+) T cells. _Cancer Lett._
500, 98–106 (2021). Article CAS PubMed Google Scholar * Liu, J. et al. Extracellular vesicle PD-L1 in reshaping tumor immune microenvironment: biological function and potential therapy
strategies. _Cell Commun. Signal._ 20, 14 (2022). Article CAS PubMed PubMed Central Google Scholar * Hsu, J. M. et al. STT3-dependent PD-L1 accumulation on cancer stem cells promotes
immune evasion. _Nat. Commun._ 9, 1908 (2018). Article PubMed PubMed Central Google Scholar * Okada, M. et al. Blockage of core fucosylation reduces cell-surface expression of PD-1 and
promotes anti-tumor immune responses of T cells. _Cell Rep._ 20, 1017–1028 (2017). Article CAS PubMed Google Scholar * Li, C. W. et al. Glycosylation and stabilization of programmed
death ligand-1 suppresses T-cell activity. _Nat. Commun._ 7, 12632 (2016). Article CAS PubMed PubMed Central Google Scholar * Zhu, L. et al. Quantification-promoted discovery of
glycosylated exosomal PD-L1 as a potential tumor biomarker. _Small methods_ 6, e2200549 (2022). Article PubMed Google Scholar * Zhu, L. et al. Coupling aptamer-based protein tagging with
metabolic glycan labeling for in situ visualization and biological function study of exosomal protein-specific glycosylation. _Angew. Chem. (Int. ed. Engl.)_ 60, 18111–18115 (2021). Article
CAS PubMed Google Scholar * Sun, W. et al. Tumor stem cell-derived exosomal microRNA-17-5p inhibits anti-tumor immunity in colorectal cancer via targeting SPOP and overexpressing PD-L1.
_Cell death Discov._ 8, 223 (2022). Article CAS PubMed PubMed Central Google Scholar * Gou, Q. et al. PD-L1 degradation pathway and immunotherapy for cancer. _Cell death Dis._ 11, 955
(2020). Article CAS PubMed PubMed Central Google Scholar * Xian, D., Niu, L., Zeng, J. & Wang, L. LncRNA KCNQ1OT1 secreted by tumor cell-derived exosomes mediates immune escape in
colorectal cancer by regulating PD-L1 Ubiquitination via MiR-30a-5p/USP22. _Front. cell developmental Biol._ 9, 653808 (2021). Article Google Scholar * Jing, H. et al. Integrin α2 promotes
immune escape in non-small-cell lung cancer by enhancing PD-L1 expression in exosomes to inhibit CD8 + T-cell activity. _J. investigative Med. : Off. Publ. Am. Federation Clin. Res._ 72,
57–66 (2024). Article Google Scholar * Alam, M. R., Rahman, M. M. & Li, Z. The link between intracellular calcium signaling and exosomal PD-L1 in cancer progression and immunotherapy.
_Genes Dis._ 11, 321–334 (2024). Article CAS PubMed Google Scholar * Wei, F. et al. Exosomal PD-L1 derived from head and neck squamous cell carcinoma promotes immune evasion by
activating the positive feedback loop of activated regulatory T cell-M2 macrophage. _Oral. Oncol._ 145, 106532 (2023). Article CAS PubMed Google Scholar * Tang, Y. et al. The biogenesis,
biology, and clinical significance of exosomal PD-L1 in cancer. _Front. Immunol._ 11, 604 (2020). Article CAS PubMed PubMed Central Google Scholar * Yang, Y. et al. Exosomal PD-L1
harbors active defense function to suppress T cell killing of breast cancer cells and promote tumor growth. _Cell Res._ 28, 862–864 (2018). Article CAS PubMed PubMed Central Google
Scholar * Xu, R. et al. Extracellular vesicles in cancer - implications for future improvements in cancer care. _Nat. Rev. Clin. Oncol._ 15, 617–638 (2018). Article CAS PubMed Google
Scholar * Chen, J. et al. GOLM1 exacerbates CD8(+) T cell suppression in hepatocellular carcinoma by promoting exosomal PD-L1 transport into tumor-associated macrophages. _Signal Transduct.
Target. Ther._ 6, 397 (2021). Article CAS PubMed PubMed Central Google Scholar * Ye, Z. et al. Manipulation of PD-L1 endosomal trafficking promotes anticancer immunity. _Adv. Sci.
(Weinh., Baden.-Wurtt., Ger.)_ 10, e2206411 (2023). Google Scholar * Shen, D. D. et al. LSD1 deletion decreases exosomal PD-L1 and restores T-cell response in gastric cancer. _Mol. cancer_
21, 75 (2022). Article CAS PubMed PubMed Central Google Scholar * Gu, H. et al. Sorting protein VPS33B regulates exosomal autocrine signaling to mediate hematopoiesis and
leukemogenesis. _J. Clin. Investig._ 126, 4537–4553 (2016). Article PubMed PubMed Central Google Scholar * Villarroya-Beltri, C. et al. ISGylation controls exosome secretion by promoting
lysosomal degradation of MVB proteins. _Nat. Commun._ 7, 13588 (2016). Article CAS PubMed PubMed Central Google Scholar * Kanemoto, S. et al. Multivesicular body formation enhancement
and exosome release during endoplasmic reticulum stress. _Biochemical biophysical Res. Commun._ 480, 166–172 (2016). Article CAS Google Scholar * Gurung, S., Perocheau, D., Touramanidou,
L. & Baruteau, J. The exosome journey: from biogenesis to uptake and intracellular signalling. _Cell Commun. Signal. : CCS_ 19, 47 (2021). Article CAS PubMed PubMed Central Google
Scholar * Liu, D. A. et al. A phosphoinositide switch mediates exocyst recruitment to multivesicular endosomes for exosome secretion. _Nat. Commun._ 14, 6883 (2023). Article CAS PubMed
PubMed Central Google Scholar * Xiang, J. et al. Exo70 Promotes the invasion of pancreatic cancer cells via the regulation of exosomes. _Cancers_ 16 (2024). * Izquierdo, E. et al.
Extracellular vesicles and PD-L1 suppress macrophages, inducing therapy resistance in TP53-deficient B-cell malignancies. _Blood_ 139, 3617–3629 (2022). Article CAS PubMed Google Scholar
* Yu, X., Harris, S. L. & Levine, A. J. The regulation of exosome secretion: a novel function of the p53 protein. _Cancer Res._ 66, 4795–4801 (2006). Article CAS PubMed Google
Scholar * Wang, J. et al. Helicobacter pylori CagA promotes immune evasion of gastric cancer by upregulating PD-L1 level in exosomes. _iScience_ 26, 108414 (2023). Article CAS PubMed
PubMed Central Google Scholar * Qiu, Y. et al. Activated T cell-derived exosomal PD-1 attenuates PD-L1-induced immune dysfunction in triple-negative breast cancer. _Oncogene_ 40, 4992–5001
(2021). Article CAS PubMed PubMed Central Google Scholar * Xu, R. et al. Aptamer-Assisted Traceless Isolation of PD-L1-Positive Small Extracellular Vesicles for Dissecting Their
Subpopulation Signature and Function. _Anal. Chem._ 95, 1016–1026 (2023). CAS PubMed Google Scholar * Wang, J. et al. Exosomal PD-L1 and N-cadherin predict pulmonary metastasis
progression for osteosarcoma patients. _J. nanobiotechnology_ 18, 151 (2020). Article CAS PubMed PubMed Central Google Scholar * Lin, W. et al. Extracellular vesicle-cell adhesion
molecules in tumours: biofunctions and clinical applications. _Cell Commun. Signal. : CCS_ 21, 246 (2023). Article PubMed PubMed Central Google Scholar * Zhang, W. et al. ICAM-1-mediated
adhesion is a prerequisite for exosome-induced T cell suppression. _Developmental cell_ 57, 329–43.e7 (2022). Article CAS PubMed PubMed Central Google Scholar * Wang, R., Yang, Y.,
Huang, J. & Yao, Y. The detection of exosomal PD-L1 in peripheral blood. _Methods Mol. Biol. (Clifton, NJ)_ 2695, 195–212 (2023). Article CAS Google Scholar * Feng, R. et al.
Cancer-associated fibroblast-derived extracellular vesicles mediate immune escape of bladder cancer via PD-L1/PD-1 expression. _Endocr. Metab. immune Disord. drug targets_ 23, 1410–1420
(2023). Article CAS PubMed Google Scholar * Yu, Z. L., Liu, J. Y. & Chen, G. Small extracellular vesicle PD-L1 in cancer: the knowns and unknowns. _NPJ Precis. Oncol._ 6, 42 (2022).
Article CAS PubMed PubMed Central Google Scholar * Ko, H. H. et al. Metastasis and immunosuppression promoted by mtDNA and PD-L1 in extracellular vesicles are reversed by WGP β-glucan
in oral squamous cell carcinoma. _Cancer Sci._ 114, 3857–3872 (2023). Article CAS PubMed PubMed Central Google Scholar * Ou, Q., Dou, X., Tang, J., Wu, P. & Pan, D. Small
extracellular vesicles derived from PD-L1-modified mesenchymal stem cell promote Tregs differentiation and prolong allograft survival. _Cell tissue Res._ 389, 465–481 (2022). Article CAS
PubMed Google Scholar * Wang, B. et al. Mutual regulation of PD-L1 immunosuppression between tumor-associated macrophages and tumor cells: a critical role for exosomes. _Cell Commun.
Signal._ 22, 21 (2024). Article CAS PubMed PubMed Central Google Scholar * Schroeder, J. C. et al. Circulating exosomes inhibit B cell proliferation and activity. _Cancers_ 12 (2020). *
Chen, Y. et al. Jianpi Yangzheng Xiaozheng decoction alleviates gastric cancer progression via suppressing exosomal PD-L1. _Front. Pharmacol._ 14, 1159829 (2023). Article CAS PubMed
PubMed Central Google Scholar * Poggio, M. et al. Suppression of Exosomal PD-L1 Induces Systemic Anti-tumor Immunity and Memory. _Cell_ 177, 414–27.e13 (2019). Article CAS PubMed PubMed
Central Google Scholar * Li, D. et al. Prostate cancer cells synergistically defend against CD8(+) T cells by secreting exosomal PD-L1. _Cancer Med._ 12, 16405–16415 (2023). Article CAS
PubMed PubMed Central Google Scholar * Ma, J., Cen, Q., Wang, Q., Liu, L. & Zhou, J. Exosomes released from PD-L1(+) tumor associated macrophages promote peritoneal metastasis of
epithelial ovarian cancer by up-regulating T cell lipid metabolism. _Biochem. biophysics Rep._ 36, 101542 (2023). CAS Google Scholar * Yin, Z. et al. Mechanisms underlying low-clinical
responses to PD-1/PD-L1 blocking antibodies in immunotherapy of cancer: a key role of exosomal PD-L1. Journal for immunotherapy of cancer. 2021;9. * Chen, G. et al. Exosomal PD-L1
contributes to immunosuppression and is associated with anti-PD-1 response. _Nature_ 560, 382–386 (2018). Article CAS PubMed PubMed Central Google Scholar * Theodoraki, M. N., Yerneni,
S. S., Hoffmann, T. K., Gooding, W. E. & Whiteside, T. L. Clinical Significance of PD-L1(+) Exosomes in Plasma of Head and Neck Cancer Patients. _Clin. Cancer Res. : Off. J. Am. Assoc.
Cancer Res._ 24, 896–905 (2018). Article CAS Google Scholar * Chen, J. et al. Tumor extracellular vesicles mediate anti-PD-L1 therapy resistance by decoying anti-PD-L1. _Cell. Mol.
Immunol._ 19, 1290–1301 (2022). Article CAS PubMed PubMed Central Google Scholar * Gong, B. et al. Secreted PD-L1 variants mediate resistance to PD-L1 blockade therapy in non-small cell
lung cancer. _J. Exp. Med._ 216, 982–1000 (2019). Article CAS PubMed PubMed Central Google Scholar * Serratì, S. et al. Circulating extracellular vesicles expressing PD1 and PD-L1
predict response and mediate resistance to checkpoint inhibitors immunotherapy in metastatic melanoma. _Mol. cancer_ 21, 20 (2022). Article PubMed PubMed Central Google Scholar *
Affolter A, et al. Modulation of PD‑L1 expression by standard therapy in head and neck cancer cell lines and exosomes. International journal of oncology. 2023;63. * Zheng, Y. et al.
Glioblastoma stem cell (GSC)-derived PD-L1-containing exosomes activates AMPK/ULK1 pathway mediated autophagy to increase temozolomide-resistance in glioblastoma. _Cell Biosci._ 11, 63
(2021). Article CAS PubMed PubMed Central Google Scholar * Rudd, C. E., Taylor, A. & Schneider, H. CD28 and CTLA-4 coreceptor expression and signal transduction. _Immunological
Rev._ 229, 12–26 (2009). Article CAS Google Scholar * Koni, M. et al. Circulating extracellular vesicles derived from tumor endothelial cells hijack the local and systemic anti-tumor
immune response: Role of mTOR/G-CSF pathway. _Pharmacol. Res._ 195, 106871 (2023). Article CAS PubMed Google Scholar * Weng, H. P. et al. Canine diffuse large b-cell lymphoma
downregulates the activity of CD8 + T-cells through tumor-derived extracellular vesicles. _Cancer cell Int._ 23, 252 (2023). Article CAS PubMed PubMed Central Google Scholar *
Ukrainskaya, V. M. et al. CAR-tropic extracellular vesicles carry tumor-associated antigens and modulate CAR T cell functionality. _Sci. Rep._ 13, 463 (2023). Article CAS PubMed PubMed
Central Google Scholar * Walker, L. S. K. The link between circulating follicular helper T cells and autoimmunity. _Nat. Rev. Immunol._ 22, 567–575 (2022). Article CAS PubMed PubMed
Central Google Scholar * Li, Z. et al. Esophageal cancer cell-derived small extracellular vesicles decrease circulating Tfh/Tfr via sEV-PDL1 to promote immunosuppression. _Cancer Immunol.,
Immunother. : CII_ 72, 4249–4259 (2023). Article CAS PubMed PubMed Central Google Scholar * Oyewole-Said, D. et al. Beyond T-Cells: Functional Characterization of CTLA-4 Expression in
Immune and Non-Immune Cell Types. _Front. Immunol._ 11, 608024 (2020). Article CAS PubMed PubMed Central Google Scholar * Liu, L. et al. Hepatic stellate cell exosome-derived circWDR25
promotes the progression of hepatocellular carcinoma via the miRNA-4474-3P-ALOX-15 and EMT axes. _Biosci. trends_ 16, 267–281 (2022). Article CAS PubMed Google Scholar * Mondal, S. K.,
Haas, D., Han, J. & Whiteside, T. L. Small EV in plasma of triple negative breast cancer patients induce intrinsic apoptosis in activated T cells. _Commun. Biol._ 6, 815 (2023). Article
CAS PubMed PubMed Central Google Scholar * Benecke, L. et al. Exosomes: Small EVs with Large Immunomodulatory Effect in Glioblastoma. International journal of molecular sciences.
2021;22. * Wang, Y. et al. Exosome CTLA-4 Regulates PTEN/CD44 Signal Pathway in Spleen Deficiency Internal Environment to Promote Invasion and Metastasis of Hepatocellular Carcinoma. _Front.
Pharmacol._ 12, 757194 (2021). Article CAS PubMed PubMed Central Google Scholar * Wolf, Y., Anderson, A. C. & Kuchroo, V. K. TIM3 comes of age as an inhibitory receptor. _Nat. Rev.
Immunol._ 20, 173–185 (2020). Article CAS PubMed Google Scholar * Cai, L., Li, Y., Tan, J., Xu, L. & Li, Y. Targeting LAG-3, TIM-3, and TIGIT for cancer immunotherapy. _J. Hematol.
Oncol._ 16, 101 (2023). Article CAS PubMed PubMed Central Google Scholar * Chiba, S. et al. Tumor-infiltrating DCs suppress nucleic acid-mediated innate immune responses through
interactions between the receptor TIM-3 and the alarmin HMGB1. _Nat. Immunol._ 13, 832–842 (2012). Article CAS PubMed PubMed Central Google Scholar * Ndhlovu, L. C. et al. Tim-3 marks
human natural killer cell maturation and suppresses cell-mediated cytotoxicity. _Blood_ 119, 3734–3743 (2012). Article CAS PubMed PubMed Central Google Scholar * Clayton, K. L. et al. T
cell Ig and mucin domain-containing protein 3 is recruited to the immune synapse, disrupts stable synapse formation, and associates with receptor phosphatases. _J. Immunol. (Baltim., Md :
1950)_ 192, 782–791 (2014). Article CAS Google Scholar * Lefebvre, A. et al. Extracellular vesicles derived from nasopharyngeal carcinoma induce the emergence of mature regulatory
dendritic cells using a galectin-9 dependent mechanism. _J. Extracell. vesicles_ 12, e12390 (2023). Article PubMed Google Scholar * de Mingo Pulido, Á. et al. The inhibitory receptor
TIM-3 limits activation of the cGAS-STING pathway in intra-tumoral dendritic cells by suppressing extracellular DNA uptake. _Immunity_ 54, 1154–67.e7 (2021). Article PubMed PubMed Central
Google Scholar * Zhang, C. X. et al. Galectin-9 promotes a suppressive microenvironment in human cancer by enhancing STING degradation. _Oncogenesis_ 9, 65 (2020). Article CAS PubMed
PubMed Central Google Scholar * Wu, J. et al. Exosomes in malignant pleural effusion from lung cancer patients impaired the cytotoxicity of double-negative T cells. _Transl. Oncol._ 27,
101564 (2023). Article CAS PubMed Google Scholar * Javadi, J. et al. Diagnostic and prognostic utility of the extracellular vesicles subpopulations present in pleural effusion.
_Biomolecules_. 11 (2021). * Li, X., Liu, Y., Yang, L., Jiang, Y. & Qian, Q. TIM-3 shuttled by MV3 cells-secreted exosomes inhibits CD4(+) T cell immune function and induces macrophage
M2 polarization to promote the growth and metastasis of melanoma cells. _Transl. Oncol._ 18, 101334 (2022). Article CAS PubMed PubMed Central Google Scholar * Cheng, Z. et al.
Tumor-derived exosomes induced M2 macrophage polarization and promoted the metastasis of osteosarcoma cells through Tim-3. _Arch. Med. Res._ 52, 200–210 (2021). Article CAS PubMed Google
Scholar * Andrews, L. P. et al. Molecular pathways and mechanisms of LAG3 in cancer therapy. _Clin. Cancer Res.: Off. J. Am. Assoc. Cancer Res._ 28, 5030–5039 (2022). Article CAS Google
Scholar * Aggarwal, V., Workman, C. J. & Vignali, D. A. A. LAG-3 as the third checkpoint inhibitor. _Nat. Immunol._ 24, 1415–1422 (2023). Article CAS PubMed PubMed Central Google
Scholar * Hao, Y. et al. Tumor-derived exosomes induce initial activation by exosomal CD19 antigen but impair the function of CD19-specific CAR T-cells via TGF-β signaling. _Front. Med._
(2023). * Yan, G. et al. Potential impact of ALKBH5 and YTHDF1 on tumor immunity in colon adenocarcinoma. _Front. Oncol._ 11, 670490 (2021). Article CAS PubMed PubMed Central Google
Scholar * Ning, J. et al. METTL3 inhibition induced by M2 macrophage-derived extracellular vesicles drives anti-PD-1 therapy resistance via M6A-CD70-mediated immune suppression in thyroid
cancer. _Cell death Differ._ 30, 2265–2279 (2023). Article CAS PubMed PubMed Central Google Scholar * Wang, J. et al. Fibrinogen-like Protein 1 Is a Major Immune Inhibitory Ligand of
LAG-3. _Cell_ 176, 334–47.e12 (2019). Article CAS PubMed Google Scholar * Maruhashi, T. et al. Binding of LAG-3 to stable peptide-MHC class II limits T cell function and suppresses
autoimmunity and anti-cancer immunity. _Immunity_ 55, 912–24.e8 (2022). Article CAS PubMed Google Scholar * Zhang, Y. et al. FGL1 in plasma extracellular vesicles is correlated with
clinical stage of lung adenocarcinoma and anti-PD-L1 response. Clinical and experimental immunology. 2023. * Kouo, T. et al. Galectin-3 Shapes Antitumor Immune Responses by Suppressing CD8+
T Cells via LAG-3 and Inhibiting Expansion of Plasmacytoid Dendritic Cells. _Cancer Immunol. Res._ 3, 412–423 (2015). Article CAS PubMed PubMed Central Google Scholar * Yakubovich, E.,
Cook, D. P., Rodriguez, G. M. & Vanderhyden, B. C. Mesenchymal ovarian cancer cells promote CD8(+) T cell exhaustion through the LGALS3-LAG3 axis. _NPJ Syst. Biol. Appl._ 9, 61 (2023).
Article CAS PubMed PubMed Central Google Scholar * Cela, I. et al. LGALS3BP is a potential target of antibody-drug conjugates in oral squamous cell carcinoma. Oral diseases. 2023. *
Mortezaee, K. & Majidpoor, J. Alternative immune checkpoints in immunoregulatory profile of cancer stem cells. _Heliyon_ 9, e23171 (2023). Article CAS PubMed PubMed Central Google
Scholar * Shi, J. et al. Molecular characteristics of single patient-derived glioma stem-like cells from primary and recurrent glioblastoma. _Anti-cancer drugs_ 33, e381–e388 (2022).
Article CAS PubMed Google Scholar * Solinas, C., Gu-Trantien, C., Willard-Gallo, K. The rationale behind targeting the ICOS-ICOS ligand costimulatory pathway in cancer immunotherapy.
_ESMO Open_. 5 (2020). * Borgeaud, M. et al. Novel targets for immune-checkpoint inhibition in cancer. _Cancer Treat. Rev._ 120, 102614 (2023). Article CAS PubMed Google Scholar * Fan,
X. et al. Exosome miR-27a-3p secreted from adipocytes targets ICOS to promote antitumor immunity in lung adenocarcinoma. _Thorac. cancer_ 11, 1453–1464 (2020). Article CAS PubMed PubMed
Central Google Scholar * Stone, E. L. et al. ICOS coreceptor signaling inactivates the transcription factor FOXO1 to promote Tfh cell differentiation. _Immunity_ 42, 239–251 (2015).
Article CAS PubMed PubMed Central Google Scholar * Sage, P. T., Francisco, L. M., Carman, C. V. & Sharpe, A. H. The receptor PD-1 controls follicular regulatory T cells in the lymph
nodes and blood. _Nat. Immunol._ 14, 152–161 (2013). Article CAS PubMed Google Scholar * Saliba, D. G. et al. Composition and structure of synaptic ectosomes exporting antigen receptor
linked to functional CD40 ligand from helper T cells. eLife. 2019;8. * Jiang, F. et al. Extracellular vesicle-derived protein file from peripheral blood predicts immune-related adverse
events in gastric cancer patients receiving immunotherapy. _Cancers_. 14 (2022). * Carthon, B. C. et al. Preoperative CTLA-4 blockade: tolerability and immune monitoring in the setting of a
presurgical clinical trial. _Clin. Cancer Res. : Off. J. Am. Assoc. Cancer Res._ 16, 2861–2871 (2010). Article CAS Google Scholar * Koyama, S. et al. Adaptive resistance to therapeutic
PD-1 blockade is associated with upregulation of alternative immune checkpoints. _Nat. Commun._ 7, 10501 (2016). Article CAS PubMed PubMed Central Google Scholar * Dulal, D. et al.
Tackling of immunorefractory tumors by targeting alternative immune checkpoints. _Cancers_ 15 (2023). * Lu, H. et al. B7-H3 confers resistance to Vγ9Vδ2 T cell-mediated cytotoxicity in human
colon cancer cells via the STAT3/ULBP2 axis. _Cancer Immunol., Immunother. : CII_ 70, 1213–1226 (2021). Article CAS PubMed Google Scholar * Purvis, I. J. et al. B7-H3 in
Medulloblastoma-Derived Exosomes; A Novel Tumorigenic Role. _Int. J. Mol. Sci_. 21 (2020). * Wu, R. et al. Exosomal B7-H3 facilitates colorectal cancer angiogenesis and metastasis through
AKT1/mTOR/VEGFA pathway. _Cell. Signal._ 109, 110737 (2023). Article CAS PubMed Google Scholar * Picarda, E., Ohaegbulam, K. C. & Zang, X. Molecular pathways: targeting B7-H3 (CD276)
for human cancer immunotherapy. _Clin. Cancer Res: Off. J. Am. Assoc. Cancer Res._ 22, 3425–3431 (2016). Article CAS Google Scholar * Joller, N., Anderson, A. C. & Kuchroo, V. K.
LAG-3, TIM-3, and TIGIT: Distinct functions in immune regulation. _Immunity_ 57, 206–222 (2024). Article CAS PubMed Google Scholar * Swatler, J. et al. 4-1BBL-containing leukemic
extracellular vesicles promote immunosuppressive effector regulatory T cells. _Blood Adv._ 6, 1879–1894 (2022). Article CAS PubMed PubMed Central Google Scholar * Holmgaard, R. B. et
al. Tumor-expressed IDO recruits and activates MDSCs in a Treg-dependent manner. _Cell Rep._ 13, 412–424 (2015). Article CAS PubMed PubMed Central Google Scholar * Akbar, S. et al.
Circulating exosomal immuno-oncological checkpoints and cytokines are potential biomarkers to monitor tumor response to anti-PD-1/PD-L1 therapy in non-small cell lung cancer patients.
_Front. Immunol._ 13, 1097117 (2022). Article CAS PubMed Google Scholar * Jung, M. Y. et al. Superinduction of immunosuppressive glioblastoma extracellular vesicles by IFN-γ through
PD-L1 and IDO1. _Neuro-Oncol. Adv._ 4, vdac017 (2022). Article Google Scholar * Ying, X., Zheng, X., Zhang, X., Yin, Y. & Wang, X. Kynurenine in IDO1(high) cancer cell-derived
extracellular vesicles promotes angiogenesis by inducing endothelial mitophagy in ovarian cancer. _J. Transl. Med._ 22, 267 (2024). Article CAS PubMed PubMed Central Google Scholar *
Burova, E. et al. Preclinical development of the anti-LAG-3 antibody REGN3767: characterization and activity in combination with the anti-PD-1 antibody cemiplimab in human PD-1xLAG-3-Knockin
mice. _Mol. Cancer Therapeutics_ 18, 2051–2062 (2019). Article CAS Google Scholar * Euvrard, R., Robert, M., Mainbourg, S., Dalle, S., Lega, J.C. Association between immune-related
adverse events and prognosis in patients treated with immune checkpoint inhibitors in melanoma: A surrogacy analysis. _Fundamental Clin. Pharmacol._ (2023). * Yoo, W. S., Ku, E. J., Lee, E.
K. & Ahn, H. Y. Incidence of endocrine-related dysfunction in patients treated with new immune checkpoint inhibitors: a meta-analysis and comprehensive review. _Endocrinol. Metab.
(Seoul., Korea)_ 38, 750–759 (2023). Article CAS Google Scholar * Vardarli, I. et al. Risk and incidence of endocrine immune related adverse effects under checkpoint inhibitor mono or
combination therapy in solid tumors: a meta-analysis of randomized controlled trials. The Journal of clinical endocrinology and metabolism. 2023. * Cristescu, R. et al. Pan-tumor genomic
biomarkers for PD-1 checkpoint blockade-based immunotherapy. _Science_ (New York, NY). 362 (2018). * Rayamajhi, S. et al. Extracellular Vesicles as Liquid Biopsy Biomarkers across the Cancer
Journey: From Early Detection to Recurrence. _Clin. Chem._ 70, 206–219 (2024). Article PubMed Google Scholar * Wang, Y. et al. Exosomal PD-L1 predicts response with immunotherapy in
NSCLC patients. _Clin. Exp. Immunol._ 208, 316–322 (2022). Article PubMed PubMed Central Google Scholar * Eslami, S. Z. et al. Circulating tumour cells and PD-L1-positive small
extracellular vesicles: the liquid biopsy combination for prognostic information in patients with metastatic non-small cell lung cancer. _Br. J. Cancer_. (2023). * Shen, B. et al. PD-L1 and
MRN synergy in platinum-based chemoresistance of head and neck squamous cell carcinoma. _Br. J. cancer_ 122, 640–647 (2020). Article CAS PubMed Google Scholar * Tran, L. et al. Cisplatin
Alters Antitumor Immunity and Synergizes with PD-1/PD-L1 Inhibition in Head and Neck Squamous Cell Carcinoma. _Cancer Immunol. Res._ 5, 1141–1151 (2017). Article CAS PubMed PubMed
Central Google Scholar * Rong, Q. X. et al. GM-CSF mediates immune evasion via upregulation of PD-L1 expression in extranodal natural killer/T cell lymphoma. _Mol. cancer_ 20, 80 (2021).
Article CAS PubMed PubMed Central Google Scholar * Li, J. W. et al. Universal extracellular vesicles and PD-L1+ extracellular vesicles detected by single molecule array technology as
circulating biomarkers for diffuse large B cell lymphoma. _Oncoimmunology_ 10, 1995166 (2021). Article PubMed PubMed Central Google Scholar * Zhang, Q., Jeppesen, D. K., Higginbotham, J.
N., Franklin, J. L. & Coffey, R. J. Comprehensive isolation of extracellular vesicles and nanoparticles. _Nat. Protoc._ 18, 1462–1487 (2023). Article CAS PubMed PubMed Central
Google Scholar * Gorgzadeh, A. et al. A state-of-the-art review of the recent advances in exosome isolation and detection methods in viral infection. _Virol. J._ 21, 34 (2024). Article
PubMed PubMed Central Google Scholar * Wang, C. et al. Nanoplasmonic Sandwich Immunoassay for Tumor-Derived Exosome Detection and Exosomal PD-L1 Profiling. _ACS Sens._ 6, 3308–3319
(2021). Article CAS PubMed PubMed Central Google Scholar * Wang, Y. et al. Rapid and sensitive detection of PD-L1 exosomes using Cu-TCPP 2D MOF as a SPR sensitizer. _Biosens.
Bioelectron._ 201, 113954 (2022). Article CAS PubMed Google Scholar * Huang, M. et al. Homogeneous, Low-volume, Efficient, and Sensitive Quantitation of Circulating Exosomal PD-L1 for
Cancer Diagnosis and Immunotherapy Response Prediction. _Angew. Chem. (Int. ed. Engl.)_ 59, 4800–4805 (2020). Article CAS PubMed Google Scholar * Lin, B. et al. Tracing Tumor-Derived
Exosomal PD-L1 by Dual-Aptamer Activated Proximity-Induced Droplet Digital PCR. _Angew. Chem. (Int. ed. Engl.)_ 60, 7582–7586 (2021). Article CAS PubMed Google Scholar * Qin, X. et al.
Simultaneous detection of cancerous exosomal miRNA-21 and PD-L1 with a sensitive dual-cycling nanoprobe. _Biosens. Bioelectron._ 216, 114636 (2022). Article CAS PubMed Google Scholar *
Chang, L. et al. Microporous PdCuB nanotag-based electrochemical aptasensor with Au@CuCl(2) nanowires interface for ultrasensitive detection of PD-L1-positive exosomes in the serum of lung
cancer patients. _J. nanobiotechnology_ 21, 86 (2023). Article CAS PubMed PubMed Central Google Scholar * Pang, Y. et al. Personalized detection of circling exosomal PD-L1 based on
Fe(3)O(4)@TiO(2) isolation and SERS immunoassay. _Biosens. Bioelectron._ 148, 111800 (2020). Article CAS PubMed Google Scholar * Fan, Z. et al. Accurate and rapid quantification of PD-L1
positive exosomes by a triple-helix molecular probe. _Analytica Chim. acta_ 1251, 340984 (2023). Article CAS Google Scholar * Erman, A. et al. The prognostic and predictive value of
human gastrointestinal microbiome and exosomal mRNA expression of PD-L1 and IFNγ for immune checkpoint inhibitors response in metastatic melanoma patients: PROTOCOL TRIAL. _Biomedicines_ 11
(2023). * Wang, J. et al. Circulating exosomal PD-L1 at initial diagnosis predicts outcome and survival of patients with osteosarcoma. _Clin. Cancer Res.: Off. J. Am. Assoc. Cancer Res._ 29,
659–666 (2023). Article CAS Google Scholar * Qiu, P., Guo, Q., Yao, Q., Chen, J. & Lin, J. Characterization of exosome-related gene risk model to evaluate the tumor immune
microenvironment and predict prognosis in triple-negative breast cancer. _Front. Immunol._ 12, 736030 (2021). Article CAS PubMed PubMed Central Google Scholar * Su, D., Zhang, Z., Xu,
Z., Xia, F. & Yan, Y. A prognostic exosome-related LncRNA risk model correlates with the immune microenvironment in liver cancer. _Front. Genet._ 13, 965329 (2022). Article CAS PubMed
PubMed Central Google Scholar * Gao, J. et al. Expression profiles and clinical value of plasma exosomal Tim-3 and Galectin-9 in non-small cell lung cancer. _Biochem. Biophys. Res.
Commun._ 498, 409–415 (2018). Article CAS PubMed Google Scholar * Zhang, P. F. et al. Cancer cell-derived exosomal circUHRF1 induces natural killer cell exhaustion and may cause
resistance to anti-PD1 therapy in hepatocellular carcinoma. _Mol. Cancer_ 19, 110 (2020). Article CAS PubMed PubMed Central Google Scholar * Liu, L. et al. MicroRNA-15a carried by
mesenchymal stem cell-derived extracellular vesicles inhibits the immune evasion of colorectal cancer cells by regulating the KDM4B/HOXC4/PD-L1 Axis. _Front. Cell Dev. Biol._ 9, 629893
(2021). Article PubMed PubMed Central Google Scholar * Chen, H. L. et al. Serum exosomal miR-16-5p functions as a tumor inhibitor and a new biomarker for PD-L1 inhibitor-dependent
immunotherapy in lung adenocarcinoma by regulating PD-L1 expression. _Cancer Med._ 11, 2627–2643 (2022). Article CAS PubMed PubMed Central Google Scholar * Shin, J. M. et al.
Sulfisoxazole elicits robust antitumour immune response along with immune checkpoint therapy by inhibiting exosomal PD-L1. _Adv. Sci. (Weinh., Baden.-Wurtt., Ger.)_ 9, e2103245 (2022).
Google Scholar * Kim, G. B. et al. Harnessing oncolytic extracellular vesicles for tumor cell-preferential cytoplasmic delivery of misfolded proteins for cancer immunotherapy. _Small
(Weinh. der Bergstr., Ger.)_ 19, e2300527 (2023). Article Google Scholar * Im, E. J. et al. Sulfisoxazole inhibits the secretion of small extracellular vesicles by targeting the endothelin
receptor A. _Nat. Commun._ 10, 1387 (2019). Article CAS PubMed PubMed Central Google Scholar * Lee, C. H. et al. Macitentan improves antitumor immune responses by inhibiting the
secretion of tumor-derived extracellular vesicle PD-L1. _Theranostics_ 12, 1971–1987 (2022). Article CAS PubMed PubMed Central Google Scholar * Ye, H. et al. In situ sprayed nanovaccine
suppressing exosomal PD-L1 by golgi apparatus disorganization for postsurgical melanoma immunotherapy. _ACS nano_ 17, 10637–10650 (2023). Article CAS PubMed Google Scholar * Lallemand,
T. et al. nSMase2 (Type 2-Neutral Sphingomyelinase) deficiency or inhibition by GW4869 reduces inflammation and atherosclerosis in Apoe(-/-) mice. _Arteriosclerosis, thrombosis, Vasc. Biol._
38, 1479–1492 (2018). Article CAS Google Scholar * Takahashi, A. et al. Exosomes maintain cellular homeostasis by excreting harmful DNA from cells. _Nat. Commun._ 8, 15287 (2017).
Article CAS PubMed PubMed Central Google Scholar * Deng, J. & Ke, H. Overcoming the resistance of hepatocellular carcinoma to PD-1/PD-L1 inhibitor and the resultant
immunosuppression by CD38 siRNA-loaded extracellular vesicles. _Oncoimmunology_ 12, 2152635 (2023). Article PubMed Google Scholar * Lee, J. & Kim, E. H. Mechanisms underlying response
and resistance to immune checkpoint blockade in cancer immunotherapy. _Front. Oncol._ 13, 1233376 (2023). Article CAS PubMed PubMed Central Google Scholar * Chang, C. et al.
Combination therapy with dendritic cell loaded-exosomes supplemented with PD-1 inhibition at different time points have superior antitumor effect in hepatocellular carcinoma. _Cancer
Immunol., Immunother. CII_ 72, 3727–3738 (2023). Article CAS PubMed Google Scholar * Veerman, R. E. et al. Antigen-loaded extracellular vesicles induce responsiveness to anti-PD-1 and
Anti-PD-L1 treatment in a checkpoint refractory melanoma model. _Cancer Immunol. Res._ 11, 217–227 (2023). Article CAS PubMed PubMed Central Google Scholar * Zhang, Y. et al. Complete
remission of tumors in mice with neoantigen-painted exosomes and anti-PD-1 therapy. _Mol. Ther.: J. Am. Soc. Gene Ther._ 31, 3579–3593 (2023). Article CAS Google Scholar * Cifuentes-Rius,
A., Desai, A., Yuen, D., Johnston, A. P. R. & Voelcker, N. H. Inducing immune tolerance with dendritic cell-targeting nanomedicines. _Nat. Nanotechnol._ 16, 37–46 (2021). Article CAS
PubMed Google Scholar * Phung, C. D. et al. Anti-CTLA-4 antibody-functionalized dendritic cell-derived exosomes targeting tumor-draining lymph nodes for effective induction of antitumor
T-cell responses. _Acta Biomater._ 115, 371–382 (2020). Article CAS PubMed Google Scholar * Liu, Y., Sun, L., Li, Y. & Holmes, C. Mesenchymal stromal/stem cell tissue source and in
vitro expansion impact extracellular vesicle protein and miRNA compositions as well as angiogenic and immunomodulatory capacities. _J. Extracell. Vesicles_ 13, e12472 (2024). Article CAS
PubMed PubMed Central Google Scholar * Zhao, L. et al. LOXL4 shuttled by tumor cells-derived extracellular vesicles promotes immune escape in hepatocellular carcinoma by activating the
STAT1/PD-L1 Axis. _Journal of immunotherapy (Hagerstown, Md : 1997)_ (2023). * Zheng, Y. et al. Identification of extracellular vesicles-transported miRNAs in Erlotinib-resistant head and
neck squamous cell carcinoma. _J. cell Commun. Signal._ 14, 389–402 (2020). Article PubMed PubMed Central Google Scholar * Yin, Y. et al. Colorectal cancer-derived small extracellular
vesicles promote tumor immune evasion by upregulating PD-L1 expression in tumor-associated macrophages. _Adv. Sci. (Weinh., Baden.-Wurtt., Ger.)_ 9, 2102620 (2022). CAS Google Scholar *
Rabe, D. C. et al. Tumor extracellular vesicles regulate macrophage-driven metastasis through CCL5. _Cancers_ 13 (2021). * Zhang, C. et al. Anti-PD-1 therapy response predicted by the
combination of exosomal PD-L1 and CD28. _Front. Oncol._ 10, 760 (2020). Article CAS PubMed PubMed Central Google Scholar * Shimada, Y. et al. Serum-derived exosomal PD-L1 expression to
predict anti-PD-1 response and in patients with non-small cell lung cancer. _Sci. Rep._ 11, 7830 (2021). Article CAS PubMed PubMed Central Google Scholar * Hao, J. et al. Homogeneous,
simple, and direct analysis of exosomal PD-L1 via aptamer-bivalent-cholesterol-anchor assembly of DNAzyme (ABCzyme) for tumor immunotherapy. _Anal. Chem._ 95, 6854–6862 (2023). Article CAS
PubMed Google Scholar Download references ACKNOWLEDGEMENTS The image editing in the article was performed with the BioRender platform (biorender.com). FUNDING This study was supported by
grants from the National Natural Science Foundation of China (82473062, 82173245, 82300881); the Sichuan Science and Technology Program (2023YFS0148 and 2023YFS0149); the Fundamental
Research Funds for the Central Universities (2022SCU12061); and the postdoctoral project, West China Hospital, Sichuan University (2023HXBH051). AUTHOR INFORMATION AUTHORS AND AFFILIATIONS *
Division of Thyroid Surgery, Department of General Surgery, West China Hospital, Sichuan University, Chengdu, China Ziyang Ye, Genpeng Li & Jianyong Lei Authors * Ziyang Ye View author
publications You can also search for this author inPubMed Google Scholar * Genpeng Li View author publications You can also search for this author inPubMed Google Scholar * Jianyong Lei View
author publications You can also search for this author inPubMed Google Scholar CONTRIBUTIONS Z.Y. wrote the manuscript in consultation with the other authors. J.L. and G.L. contributed to
the writing and editing of the manuscript. CORRESPONDING AUTHOR Correspondence to Jianyong Lei. ETHICS DECLARATIONS COMPETING INTERESTS The authors declare that the research was conducted in
the absence of any commercial or financial relationships that could be construed as potential conflicts of interest. ADDITIONAL INFORMATION PUBLISHER’S NOTE Springer Nature remains neutral
with regard to jurisdictional claims in published maps and institutional affiliations. RIGHTS AND PERMISSIONS OPEN ACCESS This article is licensed under a Creative Commons Attribution 4.0
International License, which permits use, sharing, adaptation, distribution and reproduction in any medium or format, as long as you give appropriate credit to the original author(s) and the
source, provide a link to the Creative Commons licence, and indicate if changes were made. The images or other third party material in this article are included in the article’s Creative
Commons licence, unless indicated otherwise in a credit line to the material. If material is not included in the article’s Creative Commons licence and your intended use is not permitted by
statutory regulation or exceeds the permitted use, you will need to obtain permission directly from the copyright holder. To view a copy of this licence, visit
http://creativecommons.org/licenses/by/4.0/. Reprints and permissions ABOUT THIS ARTICLE CITE THIS ARTICLE Ye, Z., Li, G. & Lei, J. Influencing immunity: role of extracellular vesicles
in tumor immune checkpoint dynamics. _Exp Mol Med_ 56, 2365–2381 (2024). https://doi.org/10.1038/s12276-024-01340-w Download citation * Received: 17 May 2024 * Revised: 06 August 2024 *
Accepted: 09 August 2024 * Published: 11 November 2024 * Issue Date: November 2024 * DOI: https://doi.org/10.1038/s12276-024-01340-w SHARE THIS ARTICLE Anyone you share the following link
with will be able to read this content: Get shareable link Sorry, a shareable link is not currently available for this article. Copy to clipboard Provided by the Springer Nature SharedIt
content-sharing initiative