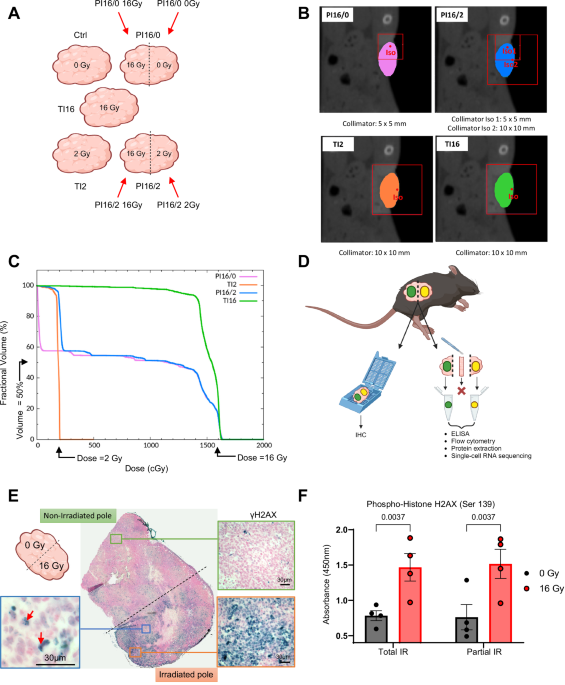
- Select a language for the TTS:
- UK English Female
- UK English Male
- US English Female
- US English Male
- Australian Female
- Australian Male
- Language selected: (auto detect) - EN
Play all audios:
ABSTRACT The efficacy and side effects of radiotherapy (RT) depend on parameters like dose and the volume of irradiated tissue. RT induces modulations of the tumor immune microenvironment
(TIME) that are dependent on the dose. Low dose RT (LDRT, i.e., single doses of 0.5–2 Gy) has been shown to promote immune infiltration into the tumor. Here we hypothesize that partial tumor
irradiation combining the immunostimulatory/non-lethal properties of LDRT with cell killing/shrinkage properties of high dose RT (HDRT) within the same tumor mass could enhance anti-tumor
responses when combined with immunomodulators. In models of colorectal and breast cancer in immunocompetent female mice, partial irradiation (PI) with millimetric precision to deliver LDRT
(2 Gy) and HDRT (16 Gy) within the same tumor induces substantial tumor control when combined with anti-PD1. Using flow cytometry, cytokine profiling and single-cell RNA sequencing, we
identify a crosstalk between the TIME of the differentially irradiated tumor volumes. PI reshapes tumor-infiltrating CD8+ T cells into more cytotoxic and interferon-activated phenotypes but
also increases the infiltration of pro-tumor neutrophils driven by CXCR2. The combination of the CXCR2 antagonist SB225002 with PD1 blockade and PI improves tumor control and mouse survival.
Our results suggest a strategy to reduce RT toxicity and improve the therapeutic index of RT and immune checkpoint combinations. SIMILAR CONTENT BEING VIEWED BY OTHERS IMMUNOTHERAPY
TARGETING DIFFERENT IMMUNE COMPARTMENTS IN COMBINATION WITH RADIATION THERAPY INDUCES REGRESSION OF RESISTANT TUMORS Article Open access 24 August 2023 BLOCKADE OF CD73 POTENTIATES
RADIOTHERAPY ANTITUMOR IMMUNITY AND ABSCOPAL EFFECTS VIA STING PATHWAY Article Open access 16 September 2024 COMBINATION RADIATION AND ΑPD-L1 ENHANCE TUMOR CONTROL BY STIMULATING CD8+ PD-1+
TCF-1+ T CELLS IN THE TUMOR-DRAINING LYMPH NODE Article Open access 14 April 2025 INTRODUCTION As a major weapon against cancer, radiotherapy (RT) is approximately used for more than 50% of
all cancer patients during their course of illness1. RT induces direct tumor cell killing through the generation of DNA damage, but RT also affects the tumor microenvironment (TME), leading
to profound changes in the anti-tumor immune response. IR induces immunogenic cell death (ICD), making tumor-associated antigens (TAAs) available for priming of tumor specific cytotoxic T
lymphocytes (CTLs)2. Moreover, IR can stimulate the interferon (IFN) cascade through the activation of the STING DNA-sensing pathway3, promoting the maturation of DCs and their antigen
presentation activity, contributing to the amplifications of an anti-tumor adaptive immune response. By triggering several features of systemic anti-tumor immunity, IR has been demonstrated
to induce anti-tumor responses in lesions outside of the irradiation field in patients4,5. This phenomenon, called “abscopal effect”, although a rare outcome, has encouraged multiple efforts
into harnessing the local and systemic effects of RT. With the emergence of immunotherapies, the interest for combining RT and immunomodulators has grown in recent years. Beneficial effects
in clinical trials have been described, notably with the use of anti-PD1 and anti-CTLA4 immune checkpoint inhibitors (ICIs)6,7,8,9,10. The ability of RT to eliminate cancer cells relies on
different technical parameters, such as the prescribed radiation dose, the distributed dose to the tumor volume and the volume of normal tissue inevitably irradiated11. However, these
parameters are also known to be critical for radiation-induced side effects. Indeed, surrounding healthy tissues still undergo cell damage eventually leading to normal tissue toxicity. These
side effects can drastically limit the therapeutic index of the treatment by inducing normal tissue injury in organs at risk in the radiation field, which may vary depending on both the
tumor localization and the type of normal tissue exposed12. Over the past decades, the delivery accuracy of the irradiation (IR) dose has greatly improved13. The development of stereotactic
body radiation therapy (SBRT) is one of the most significant advances in modern radiotherapy, and a treatment of choice in several settings14. By using accurate target delineation, motion
management, conformal treatment planning, and daily image guidance, SBRT can deliver high doses in a few fractions and provide a steep dose fall-off outside the target15. However, despite
substantial improvement in this area in recent years, radiation-related toxicities are still common, sometimes limiting the prescription of tumor curative dose to tumors close to organs at
risk when dose/volumes of normal tissue irradiated for critical organs are above tolerance thresholds. Several efforts have been made to limit radiation side effects, precision’s
radiotherapy technology developments have increased ballistic accuracy and optimized the gradient between normal tissue and tumor dose de-escalation strategies have also been proposed
conveying the potential risk of under effective tumor cell kill. Beside direct tumor cell killing, experimental approaches exploring the impact of IR dose reduction have enlightened the
interesting effects of low dose-radiation therapy (LDRT) on the TME. LDRT could enable the recruitment of tumor-reactive effector T cells, but also trigger the polarization of M2-like
macrophages into M1-like phenotypes and increase tumor vascularization16. LDRT improves TME’s immune infiltration, favoring responsiveness to combinatorial immunotherapy17. These data
suggest that low IR doses could be used to promote an RT-induced anti-tumor immune activation. In agreement, immune activation was observed in bilateral tumor settings with the primary
tumors irradiated with high dose-radiation therapy (HDRT) and the secondary tumors with LDRT in different settings18,19,20,21. In contrast to dose de-escalations, few approaches have been
tested regarding the reduction of irradiation volumes22, mainly in clinical settings23. Preliminary observations made in patients with large, advanced solid tumors suggested that the
reduction of tumor irradiated volume was not significantly affecting the efficacy of a combination of SBRT + anti-PD124, followed by a phase 1 study showing the safety of partial tumor
irradiation combined with pembrolizumab25. We hypothesized that performing image-guided partial tumor irradiations combining the immunostimulatory/non-lethal properties of LDRT with cell
killing/tumor shrinkage properties of HDRT within the same tumor mass would prompt an anti-tumor effect when combined with appropriate ICI. This modality of spatially fractionated RT (SFRT)
might offer the potential to reduce RT-related toxicities by reducing the volume of tissue irradiated at high doses and theoretically combine both the effects of optimal tumor cell killing
and TME reshaping by taking advantage of different dose response on those two compartments (i.e., the tumor and TME). In this work, we performed irradiations with millimetric precision to
deliver different IR doses within the same tumor mass in murine colorectal and breast cancer models, using female mice. We find that the combination of PD1 blockade with LDRT and HDRT within
the same tumor volume induces substantial tumor control. We decipher the influence of partial irradiation on the immune microenvironment of the differentially irradiated tumor volumes. Our
results show crosstalk between the differentially-treated areas of the tumor, notably illustrated by the increased infiltration of CD8+ T cells and neutrophils, along with their peculiar
phenotypic shifts observed in partially-irradiated tumors combining LDRT and HDRT. Single-cell RNA sequencing analysis reveals that partial irradiation with LDRT and HDRT deeply reshapes the
tumor-infiltrating CD8T cells into more cytotoxic and interferon-activated phenotypes. However, this irradiation setting also favors protumor phenotypes among tumor-infiltrating
neutrophils. In this context, we identify CXCR2 as a target to reduce protumor neutrophil infiltration following partial irradiation. We demonstrate that combining SB225002 (a potent,
selective and non-peptide CXCR2 antagonist) with PD1 blockade and partial irradiation (LDRT + HDRT) further improves tumor control and mouse survival. RESULTS THE COMBINATION OF HIGH AND LOW
IRRADIATION DOSES WITHIN THE SAME TUMOR MASS AND ANTI-PD1 ARE EFFECTIVE AGAINST MURINE COLORECTAL AND BREAST TUMORS We established experimental murine tumor models that allowed us to
perform partial tumor irradiation with millimetric precision. Immunocompetent C57BL/6 mice were injected subcutaneously with MC38 tumor cells, and the total and partial tumor irradiations
were performed using a Small Animal Radiation Research Platform (SARRP). As depicted in the schematic diagram in Fig. 1A, we performed irradiations of the whole tumor volume (total
irradiation, TI) at high dose (16 Gy, TI16) or low dose (2 Gy, TI2), or irradiations of 50% of the tumor volume (partial irradiation, PI) at 16 Gy (PI16/0), and a combination of 50% of tumor
volume irradiated at 16 Gy with the other 50% irradiated at 2 Gy (PI16/2). The techniques used to perform PI, including the combination of high and low doses (segmentations shown in Fig.
1B), showed optimal dose distributions (Fig. 1C). We biologically validated the precision and reproducibility of the PI by performing γH2AX immunohistochemistry. The tumor was oriented
thanks to a two-ink staining at the time of tumor collection (Fig. 1D) and γH2AX positivity was restricted to the target irradiated volume (Fig. 1E). The ink staining also allowed us, for
some experimental settings, to separate the two parts of PI tumors (Fig. 1D), which were subsequently analyzed independently. The reliability of such a procedure is demonstrated by γH2AX
ELISA, which showed similar levels of Ser139 phosphorylation in tissues from PI tumors when compared to their TI counterparts (Fig. 1F). We next evaluated the efficacy of the different IR
regimens in combination with anti-PD1 or control IgG, against murine colorectal (MC38 and CT26) and triple negative breast (4T1) tumors. TI at 16 Gy resulted in a strong anti-tumor response,
with complete tumor regressions in 70% of the mice bearing subcutaneous MC38 tumors and extended survival (Fig. 2A–C). In contrast, PI16/0 did not significantly slow tumor growth and did
not improve mouse survival. The combination of high dose and low dose (PI16/2) induced a slight growth delay and only weakly improved the mouse survival. When mice were treated with PD1
blocking antibodies (Supplementary Fig. 1A), non-irradiated mice and mice treated by PI16/0 showed a weak response, while the combination of HDRT and LDRT (PI16/2) with anti-PD1 exerted a
strong anti-tumor activity (Fig. 2A–C), with a significantly improved survival (median survival: 21 days for control mice, median survival was not reached in PI16/2+anti-PD1 group, Fig. 2C).
The efficacy of PI16/2 with anti-PD1 was even higher in the CT26 model with nearly 90% of the mice that had complete tumor clearances (Fig. 2D, E and Supplementary Fig. 1B). We further
validated the efficacy of intratumor dose modulation in combination with anti-PD1 in an orthotopic setting, using the poorly immunogenic 4T1 cell line. As expected, the efficacy of all
treatments involving anti-PD1 were lower than in the colorectal setting, with no efficacy of ICI alone or in combination with LDRT (Supplementary Fig. 1D, E). In contrast, PI16/2 + anti-PD1
exhibited significant anti-tumor activity and extended survival. The combination of LDRT and HDRT, but not PI16/0, sensitized 4T1 tumors to PD1 blockade, with an increased survival in PI16/2
+ anti-PD1 when compared to PI16/2 irradiation alone. THE IMMUNE ENVIRONMENT OF TUMORS PARTIALLY-IRRADIATED WITH HIGH AND LOW DOSES PRESENTS DISTINCTIVE FEATURES With the goal to further
improve the efficacy of the combination of PI with ICIs, and to investigate the mechanisms underlying the efficacy of PI16/2 combined with anti-PD1, we analyzed the modulation of the MC38
tumor immune environment induced by the different irradiation treatments. Two days after irradiation, when tumor growth curves of the different treatment groups started to diverge (Fig. 2B,
C), we collected the tumors and separated the differently irradiated tumor volumes (Fig. 3A) following the procedure described in Fig. 1D. Flow cytometry analyses showed slightly reduced
CD8+ T cells levels in TI tumors both at 2 and 16 Gy compared to control samples, which was not observed in PI tumors (Fig. 3B). Of note, in the tissue from PI16/2 that received 16 Gy, CD8+
T cell density was significantly higher than in TI16 tumors. We observed a significant increase in NK cells density in the 16 Gy volume from PI16/2 tumors, both compared to control and to
TI16 specimens (Fig. 3B). Regarding the myeloid compartment, neutrophils were increased in all volumes that received 16 Gy irradiations, both in TI and PI samples, reaching significance in
the PI16/2 sample. In the 2 Gy volume of PI16/2 tumors, neutrophil levels trended towards higher levels than in TI2, suggesting an impact of the proximity of the 16 Gy tumor volume. The
neutrophils from both volumes of PI16/2 tumors expressed high levels of immunosuppressive surface molecules such as PD-L1 and CD206 (Supplementary Fig. 2B). An increase of regulatory T cells
(Tregs) and monocytes was also observed in the 16 Gy volume of PI16/2, when compared to TI16 samples (Supplementary Fig. 1B, C). We next analyzed the cytokine/chemokine profile of the
different samples. sPLS-DA analysis using 35 cyto/chemokines as variables showed that most of the samples from 16/2 irradiated tumors were projected in the same sPLS-DA regions than the TI16
and TI2 samples respectively, but in specific sub-regions (right for PI16/2–16 Gy and top for PI16/2–2 Gy) (Fig. 3C), suggesting that PI impacted the cytokine environment differently from
TI, either at low or high IR doses. CCL5 was significantly increased in all irradiated tumor tissues, with the highest concentration observed in PI16/2 samples both in the 16 Gy and 2 Gy
volumes (Fig. 3D). The other CCR5 ligand (CCL4) was significantly increased in samples IR at 16 Gy either from PI or TI tumors. We also observed a significant increase in the CXCR2 ligand
CXCL1 in the 16 Gy volume from PI16/2 tumors (Fig. 3D). The highest levels of another CXCR2 ligand, CXCL2, were also observed in the 16 Gy volume of PI tumors. In order to deeply
characterize the phenotypic landscape of the immune cells, we performed single cell RNA sequencing on CD45+ cells from the different samples. Following unsupervised clustering, we identified
the main lymphoid and myeloid immune populations according to their gene expression profiles (Fig. 4A, B). The proportion of neutrophils strongly increased in samples irradiated at 16 Gy,
and in particular in PI tumors (Fig. 4C). Of note, in the volume irradiated at 2 Gy of PI16/2 tumors, the increase in the proportion of neutrophils was markedly higher than in TI2 tumors.
These data, together with the flow cytometry and cytokine profiling analyses, demonstrates that PI at 16/2 Gy induces deep changes in the tumor immune environment. CD8+ T CELLS ARE
PHENOTYPICALLY RE-SHAPED FOLLOWING PARTIAL IRRADIATION, AND ARE CRITICAL IN MEDIATING ITS ANTI-TUMOR ACTIVITY CD8+ T cells are known effectors of the IR-induced anti-tumor immune response,
as well as one of the main targets of ICIs as anti-PD1. Moreover, their levels were found to be particularly increased in PI16/2 tumors. We thus performed a deep characterization of their
phenotype and its modulation upon the different IR schemes. We performed an unsupervised sub-clustering of the CD8+ cluster identified by scRNA-seq, detecting six main CD8+ subpopulations
presenting distinctive expression profiles (Fig. 5A). By analyzing their transcriptomic signature, we identified each cluster as corresponding to a specific CD8+ T cell phenotype (Fig. 5C
and Supplementary Fig. 3A), including proliferating, naive, short live effector, IFN-activated, pre-memory effector and central memory CD8+ cells (Supplementary Fig. 3B). Pseudotime analysis
showed that, in our tumor model, CD8+ T cells progressed from their naïve state to an interferon (IFN)-activated phenotype, with their evolution then directed either towards memory
functions or to a short-lived fate with a phenotype presenting signs of exhaustion (Fig. 5B), including the expression of immune checkpoints as PD1 and LAG3 (Supplementary Fig. 3A). When we
analyzed the distribution of CD8+ T cells from the different samples in the six sub-clusters, we observed a strong increase in the proportion of IFN-activated cells in samples from 16/2
tumors in both the 2 Gy and 16 Gy volumes, with a concomitant reduction of the short-lived cluster, when compared to control tumors (Fig. 5C). This phenotype shift was not observed in the
other irradiated samples, except in TI2 ones but to a lower extent. In agreement with the observed shift towards an activated state, CD8+ T cells from PI16/2 tumors presented the highest
type IFN activation score, together with TI2 samples (Fig. 5D), and a similar picture was observed concerning their cytotoxicity score (Fig. 5E). These data were supported by flow cytometry
results suggesting that combining PD1 blockade to PI16/2 irradiation could increase the infiltration of CD8+ T cells expressing IFNγ and Granzyme B (Supplementary Fig. 3C), at least in the 2
Gy portion of the tumor. Of note, CD8+ T cells from the 16 Gy and 2 Gy irradiated portions of PI16/2 tumors showed very similar expression profiles in the scRNA-seq data, with few
differentially modulated genes, in contrast to the large number of differentially modulated genes identified when comparing TI16 vs. TI2 samples (compare Supplementary Fig. 3D to
Supplementary Fig. 3E). Given the specific phenotype observed in PI16/2 tumors compared to TI tumors (Fig. 5C–E) and that CD8+ T cells are targets of PD1 blocking antibodies, we sought to
determine a functional role of CD8+ T cells in the anti-tumor response to 16/2 partial irradiations, alone and in combination with anti-PD1. When we depleted CD8+ T cells, the response to
partial irradiations was completely impaired, both in terms of tumor sizes (Fig. 6A, C) and mouse survival (Fig. 6B), including in groups treated with anti-PD1 antibodies. Conversely, CD8+ T
cells were partly dispensable in TI16 irradiation settings, as we observed a delayed tumor growth and a significantly increased survival in the TI16 + anti-CD8 group, when compared to
control mice (Fig. 6A–C). Altogether, these data indicate that CD8+ T cells are pivotal mediators of the efficacy of the combination of partial irradiation and ICIs. TARGETING IMMATURE
NEUTROPHIL INFILTRATION IN PARTIALLY-IRRADIATED TUMORS THROUGH CXCR2 BLOCKADE IMPROVES THE RESPONSE TO ANTI-PD1 As for CD8+ T cells, we observed that the number and the proportion of
neutrophils were particularly elevated in PI16/2 tumors (Figs. 3B and 4C). Neutrophils can acquire different characteristics in the tumor environment, contributing either to proinflammatory
or to immunosuppressive activities. An unsupervised analysis of the neutrophil cluster identified by scRNA-seq revealed that these cells could be distinguished as three distinct groups,
corresponding to different neutrophil phenotypes (Fig. 7A and Supplementary Fig. 4A, B). Pseudotime analysis confirmed the progression of neutrophils from an immature state to intermediate
and then mature phenotype (Fig. 7B). The distribution of these three neutrophil clusters was uneven in the different irradiation groups, with a decrease of the proportion of mature
neutrophils in the PI16/2 tumors, at a particularly high extent in the 16 Gy irradiated samples from these tumors (Fig. 7C). A supervised analysis and related UMAP projections from the
different groups showed that neutrophils from the PI16/2 samples were characterized by a specific transcriptional program compared with TI counterparts, and notably when comparing TI16 to
PI16/2 16 Gy samples (Supplementary Fig. 4C), suggesting that PI16/2 neutrophils acquired a specific phenotype in these samples. The neutrophil degranulation score, recapitulating their
ability to release cytotoxic molecules that trigger the apoptotic elimination of cancer cells, was significantly reduced in PI16/2 tumors, with the lowest score observed in the tumor volume
receiving 16 Gy (Fig. 7D). Moreover, a score that indicated the T cell suppression activity of neutrophils was significantly increased in the 16 Gy volume of the PI16/2 tumors both compared
with the control group but also with the TI16 group (Supplementary Fig. 4D). These data, together with the increased levels of immunosuppressive membrane markers observed in neutrophil from
PI16/2 samples (PD-L1 and CD206, Supplementary Fig. 2B), suggested that neutrophils could contribute to immunosuppression after partial irradiations. Accordingly, their negative role in the
tumor response to partial irradiation with high and low doses of RT was confirmed using Ly6G antibodies, which depleted neutrophils (Supplementary Fig. 5A, B) and improved tumor control and
mouse survival when combined to PI16/2 and anti-PD1 (Supplementary Fig. 5C–E). Neutrophil depletion was validated using Gr-1 antibodies (Supplementary Fig. 5A, B) in order to avoid the
epitope masking of Ly6G26. Nevertheless, Ly6G expression being restricted to mice, this approach had no translational potential, and thus we sought a strategy to target the neutrophil
population with a clinically druggable target. CXCR2 is a pivotal chemokine receptor for neutrophil trafficking and its expression was found predominantly in their immature cluster
(Supplementary Fig. 4E). In agreement with an increased proportion of immature neutrophils in PI16/2 tumors (Fig. 7C), the highest CXCR2 expression levels were found in tumors partially
irradiated with 16 Gy and 2 Gy (Fig. 7E). Flow cytometry analyses showed that the neutrophils levels in PI16/2 tumors were also increased after PD1 treatment, including the subset expressing
CXCR2 (Supplementary Fig. 5F). These observations, together with the increase levels observed for the CXCR2 ligands CXCL1 (and a trend for CXCL2) in PI16/2 tumors (Fig. 3D), led us to
hypothesize that, in partially irradiated tumors, CXCR2 drove neutrophil infiltration, and particularly the ones presenting an immature phenotype. To confirm this hypothesis, we treated mice
with the selective CXCR2 antagonist SB225002 and we showed that this pharmacological intervention prevented the neutrophil increase observed in the 16 Gy volumes from PI16/2 tumors (Fig.
8A). CXCR2 blockade did not impact the infiltration of other immune cells, such as CD8+ T and NK cells that were even increased in PI16/2 16 Gy samples (Fig. 8A). When we combined PI16/2
with anti-PD1 and CXCR2 blockade with SB225002, tumor control (Fig. 8B, D) and survival (Fig. 8C) were further improved compared with control group (median survivals: control 23 days, PI16/2
+ anti-PD1 42 days, PI16/2 + anti-PD1 + SB225002: not reached as more than 50% complete responses), suggesting that the CXCR2-recruited cells exerted immunosuppressive activities. This
observation is supported by data obtained in the 4T1 orthotopic model, with the group treated with SB225002 trending towards a reduced tumor growth and a better survival, even if no complete
response was observed in this poorly immunogenic model (Supplementary Fig. 6A–C). DISCUSSION The approach that we present here, combining irradiations with millimetric precision to the
identification and collection of differently irradiated tumor volumes, allowed us to gain insights concerning the modulation of the TME of tumors that received non-homogenous IR doses. With
this approach we found that tumors that received a high IR dose on half of their volumes combined with LDRT on the other half presented a TME that was different from the tumor totally
irradiated at the same doses, in terms of cytokines, proportion of immune populations, as well as their phenotype. These specific TME modifications were associated with a synergistic
anti-tumor activity with anti-PD1. We demonstrated that the combination of partial IR (LDRT + HDRT) and PD1 blockade was able to promote complete responses in murine colorectal tumors. These
observations obtained with LDRT and HDRT performed within the same tumor mass may reflect the immune activation observed in abscopal settings where the primary tumors were irradiated with
HDRT and the secondary tumors with LDRT18,19,20, showing sign of systemic anti-tumor immune response. We selected 16 Gy as an irradiation dose that was sufficient, in a single
administration, to induce complete responses in the MC38 subcutaneous tumors. The data reported in Fig. 2A–C confirm that 70% of the mice had complete responses after a TI16 irradiation.
Moreover, the non-lethal but immunostimulatory properties of LDRT in the range of 0.5–2 Gy16,17 make the combination with HDRT an attractive strategy offering the potential to differently
impact tumor cells and the TME, taking advantage of differences in dose-response between those compartments. We validated a better efficacy of PI16/2 + anti-PD1 than TI2 + anti-PD1 in all
three different models, highlighting the crucial role of the ablative radiation dose in the PI setting. Of note, the results achieved in the CT26 tumor model were obtained with large tumors
(tumor volume on the day of irradiation of ~140–150 mm3), suggesting that also bulky tumors could benefit from such irradiation regimens in combination with IO, in particular for “hot” tumor
lesions. Further studies will be needed to define the optimal range of HDRT and LDRT to achieve a better immune activation and improve the response when combined with immunotherapy. Since
LDRT at doses of 0.5 Gy or lower are used in certain non-oncologic settings to treat inflammatory and neurodegenerative conditions due to observed anti-inflammatory effects27,28, a fine
tuning of the doses of LDRT will be required to optimize the combination with immunotherapy agents. The detailed characterization of the TME of PI tumors allowed us to further improve the
efficacy of PI16/2 with anti-PD1. In these tumors we observed, especially in the portions receiving HDRT from PI tumors that also received LDRT, a stronger infiltration of effector immune
populations such as NK and CD8+ T cells, when compared to other conditions, and in particular to TI tumors. We also observed a trend of increase in CD8+ T (~1.4 fold) cells in the
non-irradiated mass from 16/0 tumors, in line with observations made using conventional cabinet irradiations22. Of note, these flow cytometry data were shown in absolute cell counts per mg
of tumor, as proportions do not always succeed in accounting for variations in less abundant populations. In the scRNA-seq data, CD8+ T cells from PI16/2 tumors showed type I IFN-activated
and cytotoxic profiles, suggesting that partial SBRT (LDRT + HDRT) could trigger anti-tumor immunity. These transcriptomic observations are supported by the analysis of the effector markers
IFNγ and Granzyme B in CD8+ T cells, showing an increase in the number of cells positive for these markers in partially irradiated tumors, in particular after anti-PD1 treatment. Although
correlative, these data could suggest a role for activated CD8+ T cells in the anti-tumor response observed following this combined treatment, which was validated by depletion experiments.
Of interest, CD8+ T cell depletion had a limited impact after total HDRT, in agreement with the view that the anti-tumor effect of ablative doses of RT is less dependent on immune activation
than observed after PI treatments. Moreover, we also observed that CD8+ T cells from both portions of PI16/2 tumors were phenotypically less different than CD8+ T cells from distinct tumors
totally irradiated with either 16 Gy or 2 Gy, as the CD8+ T cells from two volumes from PI16/2 tumors had few differentially-expressed genes. This strongly suggests a bilateral crosstalk
between the adjacent parts of PI16/2. More generally, our results suggest that the immunological differences observed in the 2 Gy region of PI16/2 tumors compared to the untreated regions in
PI16/0 tumors could contribute to the different outcomes observed in Fig. 2A–C. These observations are supported by the fact that LDRT, when combined with HDRT and PD1 blockade, is able to
induce a synergistic abscopal effect and effector CD8+ T cell infiltration in the TME in a model of subcutaneous MC38 implanted on both flank of mice, and had also shown efficacy in a
clinical setting18,29. Hence, the immune effector response resulting from the interplay between HDRT and LDRT-treated areas and the synergy with PD1 blockade appear to be one of the armed
wings of this combined treatment. In this regard, we found that the levels of the cytokinome in the tumor are differently modulated by the different irradiation modalities. Nevertheless,
these analyses are limited to a single time point, and future studies will be needed to evaluate them with a longer kinetic, and to identify the cell types (tumor, immune, other stromal
cells) producing such cytokines. Such studies could provide informative insights concerning the modulation of TIME induced by such a heterogeneous radiation dose distribution in the tumor.
We also observed a strong infiltration of neutrophils in the TME of PI16/2 tumors, as well as a marked shift in their phenotype. As a highly plastic type of cells, neutrophils are indeed
known to be strongly influenced by their microenvironment and remain difficult to define since their function seems to evolve throughout tumor development30. However, this type of cells
could also contribute to cancer progression through various tumor promoting functions including proliferation, aggressiveness, and dissemination, as well as immune suppression31. This
ambiguous role has been illustrated by attempts to describe neutrophils subpopulations with a similar dichotomy to “M1-like”/”M2-like” macrophages; “N1-like” and “N2-like” respectively
representing pro-tumor and anti-tumor phenotypes of neutrophils. However, key genetic markers describing these phenotypic states are yet to be fully identified and this oversimplified
dichotomy mostly relies on functional and morphological differences32. Using scRNA-seq, we characterized neutrophils according to their differentiation level, as we identified “immature”
features such as _SELL_, _RETNLG_, _HP_ and _PGLYRP1_33,34. Pseudotime analysis was then used to associate neutrophil clusters with basic phenotypic characterization based on these settings.
This classification was confirmed as the later stages of the pseudotime were represented by neutrophils expressing stronger levels of _PPIA_, _PTMA_, _MIF_, _CSTB_, and _PSAP_, which are
commonly linked with more differentiated phenotypes33,35,36,37. These more differentiated phenotypes have been associated with an increase in neutrophil degranulation (release of cytotoxic
molecules that trigger the apoptotic elimination of cancer cells) and inflammation activity, which can be described as anti-tumor features33,38,39,40. In addition to the increased
infiltration of neutrophils in partially irradiated tumors combining LDRT and HDRT, scRNA-seq analysis revealed a phenotypic shift towards more “immatures” profiles. These profiles are often
associated with immunosuppressive phenotypes, which was confirmed by the higher “T cell suppression score” and PD-L1 or CD206 neutrophil expression in these treatment conditions. These
subpopulations of neutrophils are indeed able to dampen T cell activity through various mechanisms, such as abnormal vascularization, hypoxia, immune checkpoints or secretion of factors like
Arginase 2, iNOS, MMP9 or IL1041. Germann and colleagues showed in immunostaining samples from colorectal cancer (CRC) human tumors that CD8+ T cells tended to be opposed to neutrophil
localization, and to be mostly located at the border of neutrophil-infiltrated tumors41. According to their results, depletion of neutrophils could induce an increase in activated T-cell
infiltration, as well as a trend to increased numbers of total T cells41,42. In our setting, neutrophils mainly infiltrate the 16 Gy portion (which is likely well controlled due to the
ablative properties of HDRT) of the PI16/2 tumors at day 2 post-irradiation. However, considering the possible bilateral crosstalk between the two portions of these tumors, neutrophils from
the regressing HDRT region could possibly traffic to the LDRT region and continue to play an immunosuppressive role. Different strategies have been explored to reduce the infiltration of
neutrophils in the TME, including targeting of the CXCR2 pathway. CXCR1/2 receptors promote neutrophil infiltration in the TME and are also thought to be a biomarker of
immuno/radioresistance43,44. Accordingly, it has been shown that increased levels of IL-8, a human ligand of CXCR2, were associated with bad prognosis, confirming pre-existing data25. In our
model, the CXCR2 receptor was more expressed in neutrophils from partially irradiated tumors combining LDRT and HDRT, and combining an anti-PD1 treatment to this irradiation scheme further
increased the tumor levels of CXCR2+ neutrophils. Neutrophils expressing higher levels of CXCR2 are described in the literature as less “mature” as this feature is associated with a recent
bone marrow outing, which was confirmed in our scRNA-seq analysis by the expression of CXCR2, mostly localized in “immature”/”intermediate” neutrophils45. Furthermore, studies have
highlighted the potential benefits of inhibiting CXCR2-mediated neutrophil infiltration in the TME41,45,46,47,48,49,50. When we combined PI16/2 with SB225002, a potent, selective and
non-peptide CXCR2 antagonist51, we observed enhanced CD8+ T cells and NK cells infiltration in the TME in addition to the decrease in neutrophil infiltration, confirming the
immunosuppressive properties of neutrophils recruited in a CXCR2-dependent manner, which favor immune exclusion50,51. These results contribute to explaining the increased efficacy of PI16/2
+ anti-PD1 when combined with SB225002, associated with a trend for extended survival. Overall, these results suggest that CXCR2 inhibition may represent a promising approach to counteract
the RT-induced immunosuppressive activity of neutrophils, even if further studies are needed to confirm the trends for a better anti-tumor response observed in the colorectal and breast
cancer models. In line with this view, previous literature findings indicated that CXCR2 inhibitors can increase the efficacy of RT52. In a phase I clinical study combining pembrolizumab
immunotherapy and multisite SBRT (delivered to at least two distinct metastases) in patients with advanced solid tumors, Luke et al. observed a good tumor control also in patients that could
receive only partial tumor irradiations24. Recently, the same team published another article completing this study, showing that this partial irradiation setting was safe and well tolerated
by patients, providing rationale for sparing organs at risk while administering a high dose to a partial tumor volume28. While constituting a promising clinical approach (especially in the
context of oligometastatic, oligoprogressive diseases), the cellular and immune mechanisms underlying partial irradiation remained hardly explored, with only one preclinical attempt22 and
none using high-precision ballistic irradiation devices, and without tumor IR dose modulation. In view of our data, reducing the tissue volume receiving HDRT, and treating with LDRT the
remaining tumor tissue, while combining with PD1 blockade appeared to be a promising strategy. In our experimental work, CT imaging allowed the tumor to be precisely delineated in two
portions of the same volume (50% of total tumor volume) for partial irradiation settings. These two hemisphere-shaped portions were then easy to separate for further analysis. We decided to
study the immune characteristics of the differentially-treated extremities of partially irradiated tumors. For this purpose, we chose to separate tumors into three portions, as described in
Methods. The two extremities were used for analysis, while the intermediate part was discarded, to remove dose uncertainties considering the irradiated volumes. However, this “intermediate”
part could also be interesting to study in order to understand the various immune interactions of high dose gradient areas. Such analyses using spatial transcriptomics will be carried out in
future studies. These further analyses may also contribute to a better comprehension of the immune changes undergoing in tumors when SFRT techniques (i.e., GRID/lattice, minibeam and
microbeam) are used, as they deliver alternance of high doses (peaks) and low doses (valleys), with several transition areas53. Additionally, it would be conceivable to imagine that toxicity
could decrease in PI settings compared to total volume HDRT, as it is observed in the frame of SFRT54. Using PI in the clinics would allow to only target tumor areas that are distant from
the potential OARs with HDRT, and to target the rest of the tumor (which is closer to OARs) with LDRT, therefore limiting toxicity to said organs. Further studies will be performed in order
to assess the reduction of side effects of PI compared to TI in preclinical settings using orthotopic tumors, which are more suitable to perform toxicity studies than subcutaneous ones. It
seems relevant to consider optimizing the administration of the irradiation dose. Considering the recent progress in imaging, but also in radiomics, it seems now possible to take tumor
heterogeneity (e.g., hypoxia, tumor areas that are poorly infiltrated with T cells, etc.) into account for the identification of the radiation dose to administer to the different tumor areas
in various SFRT settings23,55,56,57. An approach involving the targeting of hypoxic areas has been proposed and tested in clinical settings (PArtial Tumor irradiation targeting HYpoxic
segment, PATHY)23,58. Moreover, instead of highly different IR doses as used here as a model to obtain proof of concept and to study the interplay between differently irradiated volumes,
gradients may be applied and would likely result in improved efficacy. Dose fractionation is another parameter to be considered. In this paper, we administered RT with a single dose, as it
was technically easier with the use of image-guided SBRT. Since it has been shown that fractionated RT could be more likely to synergize with ICI than single-dose RT, and induce
ICD59,60,61,62, future attempts involving fractionated partial RT may result in further improved efficacies. In conclusion, we showed that partial irradiation combining LDRT + HDRT,
associated with the blockade of PD1 and CXCR2 was a potent way to promote anti-tumor activity, leading to several complete responses. While further studies are needed to improve and optimize
partial irradiation settings, our work may open new fields to both reduce RT toxicity and to improve therapeutic index of RT and ICI combinations. Our results have translational relevance,
especially considering that CXCR2 inhibitors are already used in the clinics63,64. This approach may be tested first in clinical contexts where IR of whole tumor volume is not feasible,
i.e., when tumor masses are too large, or close to radiosensitive organs, preventing the tumoricidal high doses to the whole tumor target. METHODS STUDY APPROVAL Animal experiments were
performed in compliance with French and European regulations on the protection of animals used for scientific purposes (EC Directive 2010/63/EU and French Decree 2013–118). All experiments
were approved by the Ethics Committee CEEA26 of Gustave Roussy (approval number I-94-076-11) and #81 at IRSN (approval number E92-032-01) and authorized by the French Ministry of Research.
CELLS MC38 colon cancer cells were purchased from Kerafast. Cells were cultured with Dulbecco’s Modified Eagle Medium (DMEM, Gibco) adjusted to contain 10% fetal bovine serum (FBS), 1%
penicillin/streptomycin, 1% sodium pyruvate, 1% HEPES buffer solution, 1% non-essential amino acids (NEAA). CT26.WT (hereafter named CT26) colon carcinoma and 4T1 mammary carcinoma cells
were purchased from ATCC. Both tumor cell lines were cultured with RPMI-1640 medium (Gibco) adjusted to contain 10% FBS and 1% penicillin/streptomycin. Cells were cultured in humidified
incubators at 37 °C with 5% carbon dioxide. ANIMAL EXPERIMENTS C57BL/6 and BALB/c female mice aged 7–8 weeks were purchased from Janvier CERT (Le Genest St. Isle, France), and housed at the
Gustave Roussy animal facility (Plateforme d’Evaluation Preclinique, PFEP) or at the IRSN Animal Research and Ethics Support Group (GSEA). All animals were included in experiments after at
least 1 week of acclimatization period. 106 cells resuspended in 50 µL of phosphate-buffered saline (PBS) were subcutaneously injected into the right hind flank of each mouse. After 8 (CT26
model) or 11 (MC38 model) days, when tumors reached 140–150 (CT26) or 100–130 (MC38) mm3, the mice were randomly divided into treatment groups. 0.75.106 4T1 cells resuspended in 50 µL of PBS
were orthotopically injected in the fat pad of each BALB/c mouse. After 13 days, when 4T1 tumors reached around 100 mm3, the mice were randomly divided into treatment groups. Measures were
performed with a caliper, and tumor volume was calculated as follows: (width² × length) × 0.5. All animal experimental procedures were carried out in accordance with the Ministry of Higher
Education, Research and Innovation (MESRI) regulations with specific authorizations. Mice were maintained in specific pathogen-free facilities. They had free access to food and water. They
were housed on a 12-h light/dark cycle at a room temperature of 22 °C ± 2 °C and a relative humidity of 55% ± 15% (five animals per cage, disposable ventilated cages, Innovive, France). The
health and behavior of the mice were assessed three times per week. Mice were euthanized by cervical dislocation on the presentation of defined criteria (tumor size exceeding 1500 mm3,
advanced tumor necrosis, global behavior), and a survival time was recorded to perform a survival analysis for the treatment groups. In some cases, the size limit has been exceeded the last
day of measurement and the mice were immediately euthanized. We adhered to criteria and guidelines in all experiments. ANTIBODIES AND TREATMENTS The anti-PD1 (clone RMP1-14, BE0146), and the
rat IgG2a isotype control (clone 2A3, BE0089) were purchased from BioXcell, and administered i.p. at 10 mg/kg after SBRT and at 5 mg/kg 3/week for 2 weeks. For depletion of CD8+ T cells and
neutrophils, the anti-CD8α antibodies (5 mg/kg, BioXcell, clone 2.43, BE0061) and anti-Ly6G (10 mg/kg, BioXcell, clone 1A8, BE0075-1), respectively, were injected i.p. on days 0, 4, and 7
post SBRT. Rat IgG2b (5 mg/kg, BioXcell, clone LTF-2, BE0090) and rat IgG2a isotype control (10 mg/kg, BioXcell, clone 2A3, BE0089), respectively, were used as controls. CXCR2 inhibitor
SB225002 (10 mg/kg, MedChemExpress, HY-16711) was dissolved in a 1% dimethyl sulfoxide (DMSO), 20% polyethylene glycol 400 (PEG400), 5% polysorbate 80 (Tween 80), and 74% ddH2O51. SB225002
was administered i.p. at 10 mg/kg after SBRT, and every day for 8 days. IRRADIATION Before irradiation, mice were anesthetized with 100 mg/kg ketamine (Imalgene 1000, Merial, France) and
xylazine (Rompoun 2%, Bayer Healthcare, France) or 2.5% isoflurane. Before and after the procedure, anesthetized mice were put in a Thermacage (Datesand). Two Small Animal Radiation Research
Platforms (SARRP, XStrahl), microirradiation systems guided by cone beam computed tomography (CBCT) images, were used for irradiation of mice. CBCT images were obtained using an
uncollimated beam (20 × 20 cm), a voltage of 60 kV, a current of 0.8 mA with inherent and additional filtrations of 0.8, and 1 mm of beryllium and aluminum, respectively, with continuous
beam on and 360 stage rotation between the x-ray source and the digital flat panel detector. The 3-dimensional (3D) reconstruction images and dose planning were performed with the Muriplan
treatment planning system (TPS)65. Irradiations were performed at a voltage of 220 kV and a current of 13 mA with inherent and additional filtration of 0.8 of beryllium and 0.15 mm (with an
additional filtration of 0.105 mm on the second machine) of copper. The protocol developed by the Radiation Therapy Committee Task Group 61 and presented by the American Association of
Physicists in Medicine was followed as much as possible in order to evaluate the half value layer (0.667 mm and 0.844 mm, copper) inside of the two SARRP66. The configuration used to obtain
the half value layer has been previously described67. Two treatment plans were designed to perform partial tumor irradiations (50 % of the tumor receiving 2 Gy, and 50 % of the tumor
receiving 16 Gy): first, a dose of 2 Gy was delivered in a single fraction to the whole tumor volume, using two beams which were at 180° to each other to ensure homogeneous dose distribution
inside the tumor. A dose of 14 Gy was then administered to 50% of the tumor volume using tumor segmentation and a dose-volume histogram (DVH). For whole tumor volume irradiation, the
isocenter was placed on the tumor, and a two-beam treatment was planned to deliver the prescribed total dose to the isocenter. For partial tumor volume irradiation, the isocenter was placed
to deliver 16 Gy to 50% of the tumor volume with a two-beam treatment. In function of the size and shape of the tumor, collimators of 3 × 3, 5 × 5, or 10 × 10 mm² were used. Both beams were
at 180° to each other to ensure homogeneous dose distribution inside the tumor. The two halves of the tumor were separated following a transverse section. Examples of TPS and associated DVH
are shown in Fig. 1B, C, respectively. TUMOR SAMPLING To collect tumor samples, preserving their spatial orientation to physically recognize and separate the 16 Gy-irradiated and
non-irradiated (or 2 Gy-irradiated)-portions, an ink staining procedure was developed. Briefly, two inks with different colors were used to stain the differentially irradiated parts of the
tumor when collecting it after mouse euthanasia. This allowed an easy orientation of the tumors for histological analysis. A schematic figure of tumor sampling and orientation is shown in
Fig. 1D. To have a clear separation of the differentially irradiated volumes for flow cytometry/protein/Single-cell RNAseq analysis, an intermediate portion was removed at the interface of
both areas after ink staining, following the same transverse section. The two other volumes (corresponding to differentially irradiated tumor volumes) were then put in distinct collection
tubes. FLOW CYTOMETRY ANALYSIS C57BL/6 mice were euthanized, and MC38 tumors were collected as described in “Tumor sampling” section. Tumors were weighed, finely chopped, and dissociated
with digestive enzyme (Tumor Dissociation Kit, mouse, Miltenyi Biotec, 130-096-730) according to the manufacturer’s instructions for 30 min at 37 °C in the ThermoMixer® C (Eppendorf). Tumor
digestions were then mechanically disrupted and filtered through a 40 µm nylon filtration cell strainer (Cell Strainer 40 µm Nylon, Falcon, USA, 352340). Resulting single cells were
incubated at 4 °C with anti-CD16/32 (clone 93, BioLegend, 101319, 1/100) antibodies for 10 min. The cells were stained using the same protocols as previously described68,69, with the
following antibodies: anti-CD69 APC (clone H1.2F3, BD Biosciences, 560689, 1/200), anti-CD11b BUV395 (clone M1/70, BD Biosciences, 563553, 1/400), anti-CD8a BV421 (clone 53–6.7, BD
Biosciences, 563898, 1/400), anti-CD62L BUV737 (clone MEL-14, BD Biosciences, 612833, 1/200), anti-CD45 PerCP-Cy5.5 (clone 30-F11, BD Biosciences, 550994, 1/200), Anti-CD45 FITC (clone
30-F11, BioLegend, 103107, 1/200), anti-CD45 APC-Cy7 (clone REA737, Miltenyi Biotec, 130-110-662, 1/50), anti-Siglec F PE-CF594 (clone E50-2440, BD Biosciences, 562757, 1/100), anti-LAG3
BV650 (clone C9B7W, BioLegend, 125227, 1/50), anti-PD1 (CD279) BV605 (clone 29F.1A12, BioLegend, 135220, 1/100), anti-CD25 PE-Cy7 (clone PC61, BD Biosciences, 552880, 1/100), anti-CD4 BV510
(clone RM4-5, BioLegend, 100559, 1/400), anti-CD19 PE-CF594 (clone 1D3, BD Biosciences, 562291, 1/200), anti-NK1.1 FITC (clone PK136, BD Biosciences, 553164, 1/100), anti-Ly6G BV421 (clone
1A8, BD Biosciences, 562737, 1/400), anti-Ly6G PerCP Cy5.5 (clone REA526, Miltenyi Biotec, 130-117-500, 1/50), anti-I-A/I-E BV510 (clone 2G9, BD Biosciences, 743871, 1/200), anti-CD64 PE-Cy7
(clone X54-5/7.1, BioLegend, 139314, 1/200), anti-CD206 PerCP Cy5.5 (clone C068C2, BioLegend, 141716, 1/200), anti-CD274 BV650 (clone MIH5, BD Biosciences, 740614, 1/100), anti-Ly6C APC-Cy7
(clone AL-21, BD Biosciences, 560596, 1/100), anti-Ly6C AlexaFluor 700 (clone HK1.4, BioLegend, 128024, 1/50), anti-CD11c BV605 (clone N418, BioLegend, 117334, 1/100), anti-CD103 BV711
(clone 2E7, BioLegend, 121435, 1/100), anti-Gr1 PE (clone RB6-8C5, Thermo Fisher Scientific, 14-5931-82, 1/200), and anti-CD182 (CXCR2) PE (clone 3F10-B3, BioLegend, 163903, 1/50). To
analyze IFNγ and Granzyme-B intracellular levels, cells were stimulated for 2 h at 37 °C with PMA/ionomycin before incubation with anti-CD16/32 antibodies followed by membrane staining.
Cells were then fixed using 4% paraformaldehyde for 15 min at 4 °C and permeabilized using Perm/Wash Buffer (BD Perm/Wash) for intracellular cytokine staining. Anti-IFNγ BV786 (clone XMG1.2,
BD Horizon, 563773, 1/50) and anti-Granzyme B PE-Cy7 (clone NGZB, eBioscience, 25-8898-80, 1/200) were used for intracellular cytokine staining. All events were acquired immediately
following sample processing using an LSR Fortessa flow cytometer (BD). All flow cytometric data analysis was performed using FlowJo v10.8.1 (FlowJo, Ashland, OR, USA). The myeloid cell
gating strategy is the same as previously described68,69, and the lymphoid cell gating strategy is described in Supplementary Fig. 2A. CYTOKINE/CHEMOKINE ARRAY MC38 tumor cells were
collected, and partially irradiated tumors were separated into two distinct groups (one for each treatment condition), following the method described in “Tumor Sampling”. Tumors were weighed
and mechanically disrupted in RIPA lysis buffer (150 mM NaCl, 1% NP-40, 0.25% Na-deoxycholate, 50 mM Tris-HCl (pH 7.4), Sigma-Aldrich, USA) containing a protease inhibitor cocktail (Roche,
Switzerland, 04-693-124-001) using Biomasher disposable homogenizers (Nippi, Japan, 320102). The cytokine and chemokine concentrations in tumor tissues were profiled using the Mouse
Cytokine/Chemokine 44-Plex Discovery Assay® Array (MD44) at Eve Technologies Corporation (AB, Canada). Protein extracts were diluted to 4 μg/μL, and the multiplex immunoassay was analyzed
with the BioPlex 200 instrument (Bio-Rad, USA). Cytokine and chemokine concentrations were calculated based on the standard curve generated using the standards included in the kit. The
concentrations of the following cytokines were calculated: Eotaxin, G-CSF, GM-CSF, IFNg, IL-1a, IL-1b, IL-4, IL-5, IL-6, IL-7, IL-10, IL-12p40, IL-12p70, IL-13, IL-15, IL-17, IP-10, KC, LIF,
LIX (CXCL5), MCP-1, M-CSF, MIG, MIP-1a, MIP-1b (CCL3), MIP-2, RANTES (CCL5), TNFa, VEGF, Fractalkine, IFNb-1, IL-11, MDC, MIP-3a and TARC. A Partial Least-Squares Discriminant Analysis
(sPLS-DA) was conducted on the 35 cytokines within the dynamic range of the assay, as a supervised multivariate analysis to discriminate the different treatment groups based on their
cytokine/chemokine concentrations. More precisely, the sPLS-DA maximizes the covariance between the cytokine/chemokine covariates by constructing components (linear combinations of the
original covariates of each block) that are maximally correlated with respect to an outcome variable, here the treatments groups. In addition, the lasso penalization was included in the loss
function of the model to ensure sparsity, variable selection, and interpretability of the sPLS-DA components70. In all the analyses, leave-one-out cross validation was used to determine the
model parameters including number of components and number of features per component. All the sPLS-DA study was performed using the package “mixOmics” (version 6.18.1) in R software. IHC
AND HISTOLOGICAL ANALYSIS MC38 tumor cells were collected (15 min after RT for gH2AX staining) and spatial orientation of partially irradiated tumors was indicated with the ink staining
procedure described in “Tumor Sampling”. Tumors were kept in a 4% paraformaldehyde (PFA) solution for 24 h, paraffin embedded, and then cut into 4 µm sections. γH2AX (ser139) immunostaining
(Abcam, Ab81299) was performed using a Ventana Benchmark automaton (Ventana, Arizona, USA), and digitized using a slide scanner (NanoZoomer S60, Hamamatsu Photonics, France). SINGLE-CELL RNA
SEQUENCING AND ANALYSIS 106 MC38 cells resuspended in 50 µL of phosphate-buffered saline (PBS) were subcutaneously injected into the right hind flank of each C57BL/6 mouse. After 11 days,
when tumors reached around 100–130 mm3, the mice were randomly divided into treatment groups. Mice were then treated according to their group and 2 days after RT, mice were euthanized, and
tumor tissues collected as described above. Single-cell suspensions were prepared and CD45+ cells were positively selected using CD45 MicroBeads (Miltenyi Biotec, 130-052-301). Viability was
measured using ViaStain AOPI Staining Solution (Ozyme, CS2-0106-5ML), and cell concentration and the % CD45-positive cells were evaluated by flow cytometry using Fixable Viability Dye
eFluor™ 780 (Thermo Fisher Scientific, 65-0865-14) and CD45 FITC (clone 30-F11, BioLegend, 103107). At least 85% of cell viability and >90% of CD45+ cell was obtained for further r
encapsulation. For each sample, 10,000 cells were encapsulated using the 10x Chromium Controller system and a Chromium Next GEM Single Cell 3ʹ Dual Index Kit v3.1. Reverse transcription and
libraries were prepared according to the manufacturer’s instruction. Two independent experiments were performed including 7 experimental conditions. In total, 16 samples were included in the
study (4 replicates of unirradiated controls, 2 replicates for the other conditions). Library quantification and quality was performed using the Agilent Bioanalyzer 2100 (Agilent Genomics).
Indexed libraries were equimolary pooled and sequenced on an Illumina NovaSEq 6000 sequencing system with a minimum depth sequencing of 20,000 read pairs/cell. Single-Cell RNA-Seq outputs
were processed using the Cell Ranger software (10x Genomics). Downstream analysis was performed using the Seurat 4.0 and Monocle 3 packages in R. Seurat objects corresponding to each sample
were generated separately using the aggregated filtered barcode matrix files (Cell Ranger output), and initial quality control steps were performed to remove low-quality cells and doublets.
Cells with >10% mitochondrial reads, <200 or >3500 expressed genes, and <1000 reads were removed from the Seurat objects. Each Seurat object was identified with the associated
treatment group. Data normalization through the NormalizeData function was then processed for each Seurat object with the LogNormalize normalization method. Biologically relevant variable
genes were then identified with the FindVariableFeatures function for each Seurat object, using the vst selection method (nfeatures = 2000). Selected features from each Seurat object were
then scaled and centered using the ScaleData function. For each treatment condition, replicate samples were then merged together. Normalization and scaling were then applied to the Seurat
objects representing each treatment condition with the same parameters as previously described. Seurat objects were then randomly downsampled with a threshold of 15,066 cells for each
treatment condition. A global Seurat object was then generated, normalized, and scaled, combining all treatment groups. Using the 2000 variable genes thus identified as input, a
principal-component analysis (PCA) was performed, and the top 30 principal components were retained for further Uniform manifold approximation and projection (UMAP) visualizations.
Subsequent cell clustering using the Louvain algorithm and visualization of the cells grouped by treatment condition were performed. We used conventional functions in Seurat FindMarkers and
FeaturePlot to identify and locate differentially-expressed genes (DEGs). These DEGs were determined by a nonparametric Wilcoxon test, and ranked based on the avglog2(FC), with a cutoff of
adjusted _p_ value < 0.05. The different cell types were identified within the Seurat clusters/groups of clusters, according to the literature and the DEGs. In order to identify
subpopulations among different cell types, associated clusters were isolated and integrated into a new Seurat object. After data normalization and scaling, a PCA was performed with the
previously described settings. Neutrophils and CD8+ T cells UMAPs were respectively generated using a Louvain resolution of 0.2 and 0.3. Subpopulations were identified using specific markers
and literature, as indicated in Supplementary Figs. 3 and 433,71,72. Heatmaps were generated using the DoHeatmap function, with a downsample _n_ = 100 and specific functional scores were
calculated using the AddModuleScore function, which calculates module scores for feature expression programs. AddModuleScore results were shown using the function ggplot, associated with the
functions geom_boxplot and geom_violin. Libraries from the Gene Ontology platform were used to generate the different module scores (GO:0001916 for the “positive regulation of T-cell
mediated cytotoxicity” score, GO:0060340 for the “positive regulation of type I interferon-mediated signaling pathway”, GO:0043315 for the “positive regulation of neutrophil degranulation”).
“T cell suppression” score was generated using key markers of T cell suppression in neutrophils41. To visualize DEGs between treatment conditions, volcano plots were generated using the
ggplot and geom_point functions. Genes with >0.4 avglog2(FC) and an adjusted _p_ value of 0.05 are labeled and highlighted in red (upregulated) or blue (downregulated). To generate
Pseudotimes, neutrophils and CD8+ T cells were isolated from the global Seurat object into independent Seurat objects as described above, and were injected into Monocle3 package73,74. Cells
were clustered following Leiden community detection using cluster_cells function, with k = 50 nearest neighbors used to create clustering graphs. Gene expression trajectories were learnt
with the learn_graph function, with a maximum number of nearest neighbors to compute in the reversed graph embedding = nn.k = 50. The function graph_test was then used, in order to identify
differentially-expressed genes across the trajectory, sorted by their Moran’s I. The expression family function used for the test was “negbinomial”, and 4 cores were used while testing each
gene for differential expression. For specific supervised analysis of neutrophils, a UMAP was performed to improve readability and interpretability of the data75. UMAP is a nonlinear
dimension reduction technique considered now as a gold standard for dimension reduction of single cell data. While UMAP is often used in literature in its unsupervised formulation, its
algorithm offers significant flexibility allowing it to be extended to include categorical label information to do supervised dimension reduction. This supervised UMAP appears particularly
appealing here to enhance the structure of the embeddings according to the numerous different types of RT treatments. This ensures a better separation between the different labels while
maintaining close those producing similar transcriptomic profiles. The supervised UMAP was performed using the UWOT (version 0.1.11) R software package76. ELISA MC38 tumor cells were
collected, and partially irradiated tumors were separated into two distinct groups (one for each treatment condition), following the method described in “Tumor Sampling”. H2AX
phosphorylation was evaluated using the PathScan® Phospho-Histone H2A.X (Ser139) Sandwich ELISA Kit (Cell Signaling Technology, 50929C). STATISTICAL ANALYSES Data are represented as the mean
± standard error of the mean (s.e.m.) or standard deviation (s.d.) as indicated in the figure legends. Normality was evaluated by the Shapiro–Wilk test. When more than two groups with
gaussian distribution were compared, one-way ANOVA with Sidak’s multiple comparisons was used or Kruskal–Wallis with Dunn’s test for multiple comparisons as indicated in the figure legends.
ROUT test with _Q_ = 2% was used to identify outliers. Two-way ANOVA was used for γH2AX staining analysis. Log-rank Mantel–Cox analyses were performed for survival curve comparisons, with no
correction made for multiple comparisons. All statistical tests were performed using GraphPad Prism software v.10 (GraphPad Software). In all types of statistical analysis values of _p_
< 0.05 were considered significant (*_p_ < 0.05; **_p_ < 0.01; ***_p_ < 0.001; ****_p_ < 0.0001). REPORTING SUMMARY Further information on research design is available in the
Nature Portfolio Reporting Summary linked to this article. DATA AVAILABILITY The scRNA-seq datasets generated in this study have been deposited in the Gene Expression Omnibus (GEO) under
accession code GSE262699. The remaining data are available within the Article, Supplementary Information or Source Data file. Source data are provided with this paper. CODE AVAILABILITY All
analytical codes to reproduce scRNA-seq figures are available at https://doi.org/10.5281/zenodo.13684101. All software used in this study are publicly available and are described in the
“Methods” section. REFERENCES * Nishiga, Y. et al. Radiotherapy in combination with CD47 blockade elicits a macrophage-mediated abscopal effect. _Nat. Cancer_ 3, 1351–1366 (2022). Article
CAS PubMed PubMed Central Google Scholar * Mondini, M., Levy, A., Meziani, L., Milliat, F. & Deutsch, E. Radiotherapy–immunotherapy combinations—perspectives and challenges. _Mol.
Oncol._ 14, 1529–1537 (2020). Article PubMed PubMed Central Google Scholar * Galluzzi, L. et al. Molecular mechanisms of cell death: recommendations of the Nomenclature Committee on Cell
Death 2018. _Cell Death Differ._ 25, 486–541 (2018). Article PubMed PubMed Central Google Scholar * McLaughlin, M. et al. Inflammatory microenvironment remodelling by tumour cells after
radiotherapy. _Nat. Rev. Cancer_ 20, 203–217 (2020). Article CAS PubMed Google Scholar * De Martino, M., Daviaud, C. & Vanpouille-Box, C. Radiotherapy: an immune response modifier
for immuno-oncology. _Semin. Immunol._ 52, 101474 (2021). Article PubMed Google Scholar * Golden, E. B. et al. Local radiotherapy and granulocyte-macrophage colony-stimulating factor to
generate abscopal responses in patients with metastatic solid tumours: a proof-of-principle trial. _Lancet Oncol._ 16, 795–803 (2015). Article CAS PubMed Google Scholar * Voorwerk, L. et
al. Immune induction strategies in metastatic triple-negative breast cancer to enhance the sensitivity to PD-1 blockade: the TONIC trial. _Nat. Med._ 25, 920–928 (2019). Article CAS
PubMed Google Scholar * Postow, M. A. et al. A prospective, phase 1 trial of nivolumab, ipilimumab, and radiotherapy in patients with advanced melanoma. _Clin. Cancer Res._ 26, 3193–3201
(2020). Article CAS PubMed PubMed Central Google Scholar * Altorki, N. K. et al. Neoadjuvant durvalumab with or without stereotactic body radiotherapy in patients with early-stage
non-small-cell lung cancer: a single-centre, randomised phase 2 trial. _Lancet Oncol._ 22, 824–835 (2021). Article CAS PubMed Google Scholar * Spurr, L. F. et al. Highly aneuploid
non-small cell lung cancer shows enhanced responsiveness to concurrent radiation and immune checkpoint blockade. _Nat. Cancer_ 3, 1498–1512 (2022). Article CAS PubMed Google Scholar *
Konopacka, M., Rogoliński, J., Sochanik, A. & Ślosarek, K. Can high dose rates used in cancer radiotherapy change therapeutic effectiveness? _Contemp. Oncol._ 20, 449–452 (2016). CAS
Google Scholar * Barazzuol, L., Coppes, R. P. & van Luijk, P. Prevention and treatment of radiotherapy-induced side effects. _Mol. Oncol._ 14, 1538–1554 (2020). Article PubMed PubMed
Central Google Scholar * Begg, A. C., Stewart, F. A. & Vens, C. Strategies to improve radiotherapy with targeted drugs. _Nat. Rev. Cancer_ 11, 239–253 (2011). Article CAS PubMed
Google Scholar * Baskar, R., Lee, K. A., Yeo, R. & Yeoh, K.-W. Cancer and radiation therapy: current advances and future directions. _Int. J. Med. Sci._ 9, 193–199 (2012). Article
PubMed PubMed Central Google Scholar * Sebastian, N. T., Xu-Welliver, M. & Williams, T. M. Stereotactic body radiation therapy (SBRT) for early stage non-small cell lung cancer
(NSCLC): contemporary insights and advances. _J. Thorac. Dis._ 10, S2451–S2464 (2018). Article PubMed PubMed Central Google Scholar * Klug, F. et al. Low-dose irradiation programs
macrophage differentiation to an iNOS+/M1 phenotype that orchestrates effective T cell immunotherapy. _Cancer Cell_ 24, 589–602 (2013). Article CAS PubMed Google Scholar * Herrera, F. G.
et al. Low-dose radiotherapy reverses tumor immune desertification and resistance to immunotherapy. _Cancer Discov._ 12, 108–133 (2022). Article CAS PubMed Google Scholar * Yin, L. et
al. Effect of low-dose radiation therapy on abscopal responses to hypofractionated radiation therapy and anti-PD-1 in mice and patients with non-small cell lung cancer. _Int. J. Radiat.
Oncol. Biol. Phys._ 108, 212–224 (2020). Article ADS PubMed Google Scholar * Barsoumian, H. B. et al. Low-dose radiation treatment enhances systemic antitumor immune responses by
overcoming the inhibitory stroma. _J. Immunother. Cancer_ 8, e000537 (2020). Article PubMed PubMed Central Google Scholar * Patel, R. R. et al. High-dose irradiation in combination with
non-ablative low-dose radiation to treat metastatic disease after progression on immunotherapy: results of a phase II trial. _Radiother. Oncol._ 162, 60–67 (2021). Article CAS PubMed
Google Scholar * Carlson, P. M. et al. Radiation to all macroscopic sites of tumor permits greater systemic antitumor response to in situ vaccination. _J. Immunother. Cancer_ 11, e005463
(2023). Article PubMed PubMed Central Google Scholar * Markovsky, E. et al. An antitumor immune response is evoked by partial-volume single-dose radiation in 2 murine models. _Int. J.
Radiat. Oncol. Biol. Phys._ 103, 697–708 (2019). Article PubMed Google Scholar * Tubin, S., Popper, H. H. & Brcic, L. Novel stereotactic body radiation therapy (SBRT)-based partial
tumor irradiation targeting hypoxic segment of bulky tumors (SBRT-PATHY): improvement of the radiotherapy outcome by exploiting the bystander and abscopal effects. _Radiat. Oncol._ 14, 21
(2019). Article PubMed PubMed Central Google Scholar * Luke, J. J. et al. Safety and clinical activity of pembrolizumab and multisite stereotactic body radiotherapy in patients with
advanced solid tumors. _J. Clin. Oncol._ 36, 1611–1618 (2018). Article CAS PubMed PubMed Central Google Scholar * Korpics, M. C. et al. Partial tumor irradiation plus pembrolizumab in
treating large advanced solid tumor metastases. _J. Clin. Invest._ 133, e162260 (2023). Article CAS PubMed PubMed Central Google Scholar * Boivin, G. et al. Durable and controlled
depletion of neutrophils in mice. _Nat. Commun._ 11, 2762 (2020). Article ADS CAS PubMed PubMed Central Google Scholar * Donaubauer, A.-J. et al. Low dose radiation therapy,
particularly with 0.5 Gy, improves pain in degenerative joint disease of the fingers: results of a retrospective analysis. _Int. J. Mol. Sci._ 21, 5854 (2020). Article CAS PubMed PubMed
Central Google Scholar * Donaubauer, A.-J. et al. Low dose radiation therapy induces long-lasting reduction of pain and immune modulations in the peripheral blood—interim analysis of the
IMMO-LDRT01 trial. _Front. Immunol._ 12, 740742 (2021). Article CAS PubMed PubMed Central Google Scholar * Menon, H. et al. Influence of low-dose radiation on abscopal responses in
patients receiving high-dose radiation and immunotherapy. _J. Immunother. Cancer_ 7, 237 (2019). Article PubMed PubMed Central Google Scholar * Ng, L. G., Ostuni, R. & Hidalgo, A.
Heterogeneity of neutrophils. _Nat. Rev. Immunol._ 19, 255–265 (2019). Article CAS PubMed Google Scholar * Németh, T., Sperandio, M. & Mócsai, A. Neutrophils as emerging therapeutic
targets. _Nat. Rev. Drug Discov._ 19, 253–275 (2020). Article PubMed Google Scholar * Zhang, Y., Guoqiang, L., Sun, M. & Lu, X. Targeting and exploitation of tumor-associated
neutrophils to enhance immunotherapy and drug delivery for cancer treatment. _Cancer Biol. Med._ 17, 32–43 (2020). Article CAS PubMed PubMed Central Google Scholar * Calcagno, D. M. et
al. SiglecF(HI) marks late‐stage neutrophils of the infarcted heart: a single‐cell transcriptomic analysis of neutrophil diversification. _J. Am. Heart Assoc._ 10, e019019 (2021). Article
CAS PubMed PubMed Central Google Scholar * Ruiz-Fernández de Córdoba, B. et al. Tumor ENPP1 (CD203a)/haptoglobin axis exploits myeloid-derived suppressor cells to promote
post-radiotherapy local recurrence in breast cancer. _Cancer Discov._ 12, 1356–1377 (2022). Article PubMed PubMed Central Google Scholar * Roth, S., Solbach, W. & Laskay, T. IL-16
and MIF: messengers beyond neutrophil cell death. _Cell Death Dis._ 7, e2049 (2016). Article CAS PubMed PubMed Central Google Scholar * Schindler, L., Smyth, L. C. D., Bernhagen, J.,
Hampton, M. B. & Dickerhof, N. Macrophage migration inhibitory factor (MIF) enhances hypochlorous acid production in phagocytic neutrophils. _Redox Biol._ 41, 101946 (2021). Article CAS
PubMed PubMed Central Google Scholar * Flerlage, T. et al. Single cell transcriptomics identifies distinct profiles in pediatric acute respiratory distress syndrome. _Nat. Commun._ 14,
3870 (2023). Article ADS CAS PubMed PubMed Central Google Scholar * Ustyanovska Avtenyuk, N., Visser, N., Bremer, E. & Wiersma, V. R. The neutrophil: the underdog that packs a
punch in the fight against cancer. _Int. J. Mol. Sci._ 21, 7820 (2020). Article PubMed PubMed Central Google Scholar * van Leent, M. M. T. et al. Prosaposin mediates inflammation in
atherosclerosis. _Sci. Transl. Med._ 13, eabe1433 (2021). Article PubMed PubMed Central Google Scholar * Contini, C. et al. Characterization of cystatin B interactome in saliva from
healthy elderly and Alzheimer’s disease patients. _Life_ 13, 748 (2023). Article ADS CAS PubMed PubMed Central Google Scholar * Germann, M. et al. Neutrophils suppress
tumor‐infiltrating T cells in colon cancer via matrix metalloproteinase‐mediated activation of TGFβ. _EMBO Mol. Med._ 12, e10681 (2020). Article CAS PubMed Google Scholar * Faget, J. et
al. Neutrophils and snail orchestrate the establishment of a pro-tumor microenvironment in lung cancer. _Cell Rep._ 21, 3190–3204 (2017). Article CAS PubMed Google Scholar * Baggiolini,
M., Walz, A. & Kunkel, S. L. Neutrophil-activating peptide-1/interleukin 8, a novel cytokine that activates neutrophils. _J. Clin. Invest._ 84, 1045–1049 (1989). Article CAS PubMed
PubMed Central Google Scholar * Hua, Q. et al. IL-8 is involved in radiation therapy resistance of esophageal squamous cell carcinoma via regulation of PCNA. _Arch. Biochem. Biophys._ 676,
108158 (2019). Article CAS PubMed Google Scholar * Jablonska, J., Wu, C.-F., Andzinski, L., Leschner, S. & Weiss, S. CXCR2-mediated tumor-associated neutrophil recruitment is
regulated by IFN-β. _Int. J. Cancer_ 134, 1346–1358 (2014). Article CAS PubMed Google Scholar * Acharyya, S. et al. A CXCL1 paracrine network links cancer chemoresistance and metastasis.
_Cell_ 150, 165–178 (2012). Article CAS PubMed PubMed Central Google Scholar * De Filippo, K. & Rankin, S. M. CXCR4, the master regulator of neutrophil trafficking in homeostasis
and disease. _Eur. J. Clin. Invest._ 48, e12949 (2018). Article PubMed PubMed Central Google Scholar * Han, X. et al. CXCR2 expression on granulocyte and macrophage progenitors under
tumor conditions contributes to mo-MDSC generation via SAP18/ERK/STAT3. _Cell Death Dis._ 10, 1–15 (2019). ADS Google Scholar * Purohit, A. et al. Host Cxcr2-dependent regulation of
pancreatic cancer growth, angiogenesis, and metastasis. _Am. J. Pathol._ 191, 759–771 (2021). Article CAS PubMed PubMed Central Google Scholar * Leslie, J. et al. CXCR2 inhibition
enables NASH-HCC immunotherapy. _Gut_ 71, 2093–2106 (2022). Article CAS PubMed Google Scholar * Cheng, Y. et al. Targeting CXCR2 inhibits the progression of lung cancer and promotes
therapeutic effect of cisplatin. _Mol. Cancer_ 20, 62 (2021). Article CAS PubMed PubMed Central Google Scholar * Liu, X. et al. Antitumor and radiosensitization effects of a CXCR2
inhibitor in nasopharyngeal carcinoma. _Front. Cell Dev. Biol._ 9, 689613 (2021). Article PubMed PubMed Central Google Scholar * Lu, Q. et al. Combining spatially fractionated radiation
therapy (SFRT) and immunotherapy opens new rays of hope for enhancing therapeutic ratio. _Clin. Transl. Radiat. Oncol._ 44, 100691 (2024). CAS PubMed Google Scholar * Mahmoudi, F.,
Shahbazi-Gahrouei, D. & Chegeni, N. The role of the spatially fractionated radiation therapy in the management of advanced bulky tumors. _Pol. J. Med. Phys. Eng._ 27, 123–135 (2021).
Article Google Scholar * Sun, R. et al. A radiomics approach to assess tumour-infiltrating CD8 cells and response to anti-PD-1 or anti-PD-L1 immunotherapy: an imaging biomarker,
retrospective multicohort study. _Lancet Oncol._ 19, 1180–1191 (2018). Article CAS PubMed Google Scholar * Tan, L. T. et al. Image-guided adaptive radiotherapy in cervical cancer.
_Semin. Radiat. Oncol._ 29, 284–298 (2019). Article Google Scholar * Kopecka, J. et al. Hypoxia as a driver of resistance to immunotherapy. _Drug Resist. Updat._ 59, 100787 (2021). Article
CAS PubMed Google Scholar * Tubin, S. et al. Shifting the immune-suppressive to predominant immune-stimulatory radiation effects by SBRT-PArtial tumor irradiation targeting HYpoxic
segment (SBRT-PATHY). _Cancers_ 13, 50 (2020). Article PubMed PubMed Central Google Scholar * Dewan, M. Z. et al. Fractionated but not single-dose radiotherapy induces an immune-mediated
abscopal effect when combined with anti-CTLA-4 antibody. _Clin. Cancer Res._ 15, 5379–5388 (2009). Article ADS CAS PubMed PubMed Central Google Scholar * Weichselbaum, R. R., Liang,
H., Deng, L. & Fu, Y.-X. Radiotherapy and immunotherapy: a beneficial liaison? _Nat. Rev. Clin. Oncol._ 14, 365–379 (2017). Article CAS PubMed Google Scholar * Chen, Y., Gao, M.,
Huang, Z., Yu, J. & Meng, X. SBRT combined with PD-1/PD-L1 inhibitors in NSCLC treatment: a focus on the mechanisms, advances, and future challenges. _J. Hematol. Oncol._ 13, 105 (2020).
Article CAS PubMed PubMed Central Google Scholar * Ashrafizadeh, M. et al. Abscopal effect in radioimmunotherapy. _Int. Immunopharmacol._ 85, 106663 (2020). Article CAS PubMed
Google Scholar * Teijeira, Á. et al. CXCR1 and CXCR2 chemokine receptor agonists produced by tumors induce neutrophil extracellular traps that interfere with immune cytotoxicity. _Immunity_
52, 856–871.e8 (2020). Article CAS PubMed Google Scholar * Sitaru, S., Budke, A., Bertini, R. & Sperandio, M. Therapeutic inhibition of CXCR1/2: where do we stand? _Intern. Emerg.
Med_. 1–18 https://doi.org/10.1007/s11739-023-03309-5 (2023). * Cho, N. & Kazanzides, P. A Treatment planning system for the small animal radiation research platform (SARRP) based on 3D
slicer. _MIDAS J_. https://doi.org/10.54294/hsb0g8 (2012). * Ma, C.-M. et al. AAPM protocol for 40-300 kV x-ray beam dosimetry in radiotherapy and radiobiology. _Med. Phys._ 28, 868–893
(2001). Article CAS PubMed Google Scholar * Dos Santos, M., Paget, V., Trompier, F., Gruel, G. & Milliat, F. Dosimetry for cell irradiation using orthovoltage (40-300 kV) X-ray
facilities. _J. Vis. Exp._ https://doi.org/10.3791/61645 (2021). * Hamon, P. et al. TGFβ receptor inhibition unleashes interferon-β production by tumor-associated macrophages and enhances
radiotherapy efficacy. _J. Immunother. Cancer_ 10, e003519 (2022). Article PubMed PubMed Central Google Scholar * De Thoré, M. G., Meziani, L., Deutsch, E. & Mondini, M. Chapter
2—Cytofluorometric characterization of the myeloid compartment of irradiated mouse tumors. in _Methods in Cell Biology_, Vol. 174 (eds Kraynak, J., Galluzzi, L., Marciscano, A. E. &
Sato, A.) 17–30 (Academic Press, 2023). * Lê Cao, K.-A., Rossouw, D., Robert-Granié, C. & Besse, P. A sparse PLS for variable selection when integrating omics data. _Stat. Appl. Genet.
Mol. Biol._ 7, 35 (2008). Article MathSciNet PubMed Google Scholar * Arsenio, J. et al. Early specification of CD8+ T lymphocyte fates during adaptive immunity revealed by single-cell
gene-expression analyses. _Nat. Immunol._ 15, 365–372 (2014). Article CAS PubMed PubMed Central Google Scholar * Shlesinger, D. et al. Single-cell immune repertoire sequencing of B and
T cells in murine models of infection and autoimmunity. _Genes Immun._ 23, 183–195 (2022). Article CAS PubMed PubMed Central Google Scholar * Trapnell, C. et al. The dynamics and
regulators of cell fate decisions are revealed by pseudotemporal ordering of single cells. _Nat. Biotechnol._ 32, 381–386 (2014). Article CAS PubMed PubMed Central Google Scholar * Qiu,
X. et al. Reversed graph embedding resolves complex single-cell trajectories. _Nat. Methods_ 14, 979–982 (2017). Article CAS PubMed PubMed Central Google Scholar * McInnes, L., Healy,
J., Saul, N. & Großberger, L. UMAP: uniform manifold approximation and projection. _J. Open Source Softw._ 3, 861 (2018). Article Google Scholar * Melville, J. uwot: The Uniform
Manifold Approximation and Projection (UMAP) Method for Dimensionality Reduction_. R package version 0.1.16, https://CRAN.R-project.org/package=uwot (2023). Download references
ACKNOWLEDGEMENTS This work has received financial support Canceropole IdF (projet Emergence) to M.M., INSERM and SIRIC SOCRATE to E.D., the Fondation ARC pour la Recherche sur le Cancer
(projet fondation ARC) to M.M., the French National Research Agency within the FRANCE2030 investment plan (grant application No. ANR-21-RHU5-0005) to E.D., The French National Cancer
Institute (INCa) under the AAP SEQ-RTH22, project INCa_16861 to F.M. and M.M.; and under the AAP PLBIO-2024, projet INCa_19441 to F.M. and M.M. We thank the staff of the animal facilities at
IRSN and GR for invaluable expertise, P. Rameau, and C. Catelain at the PFIC platform at Gustave Roussy, and P. Gonin and K. Ser-Le-Roux at the PFEP platform at Gustave Roussy. AUTHOR
INFORMATION Author notes * These authors contributed equally: Fabien Milliat, Eric Deutsch. AUTHORS AND AFFILIATIONS * Gustave Roussy, INSERM U1030, Université Paris-Saclay, Villejuif,
France Paul Bergeron, Lisa Sitterle, Winchygn Liu, Marine Gerbé de Thoré, Céline Clémenson, Lydia Meziani, Cathyanne Schott, Giulia Mazzaschi, Kevin Berthelot, Eric Deutsch & Michele
Mondini * Institut de Radioprotection et de Sûreté Nucléaire (IRSN), PSE-SANTE/SERAMED/LRAcc, Fontenay-aux-Roses, France Morgane Dos Santos * Institut de Radioprotection et de Sûreté
Nucléaire (IRSN), PSE-SANTE/SERAMED/LRMed, Fontenay-aux-Roses, France Georges Tarlet, Jeremy Lavigne & Fabien Milliat * Institut de Radioprotection et de Sûreté Nucléaire (IRSN),
PSE-SANTE/SERAMED, Fontenay-aux-Roses, France Mohamed Amine Benadjaoud Authors * Paul Bergeron View author publications You can also search for this author inPubMed Google Scholar * Morgane
Dos Santos View author publications You can also search for this author inPubMed Google Scholar * Lisa Sitterle View author publications You can also search for this author inPubMed Google
Scholar * Georges Tarlet View author publications You can also search for this author inPubMed Google Scholar * Jeremy Lavigne View author publications You can also search for this author
inPubMed Google Scholar * Winchygn Liu View author publications You can also search for this author inPubMed Google Scholar * Marine Gerbé de Thoré View author publications You can also
search for this author inPubMed Google Scholar * Céline Clémenson View author publications You can also search for this author inPubMed Google Scholar * Lydia Meziani View author
publications You can also search for this author inPubMed Google Scholar * Cathyanne Schott View author publications You can also search for this author inPubMed Google Scholar * Giulia
Mazzaschi View author publications You can also search for this author inPubMed Google Scholar * Kevin Berthelot View author publications You can also search for this author inPubMed Google
Scholar * Mohamed Amine Benadjaoud View author publications You can also search for this author inPubMed Google Scholar * Fabien Milliat View author publications You can also search for this
author inPubMed Google Scholar * Eric Deutsch View author publications You can also search for this author inPubMed Google Scholar * Michele Mondini View author publications You can also
search for this author inPubMed Google Scholar CONTRIBUTIONS Study design: F.M., E.D., and M.M. Methodology, acquisition of data and analysis of data: P.B., M.D.S., L.S., G.T., J.L., W.L.,
M.G.d.T., C.C., L.M., C.S., G.M., K.B., M.A.B., F.M., and M.M.; writing of the manuscript: P.B. and M.M.; review and edition of the manuscript: P.B., M.D.S., L.S., G.T., J.L., W.L.,
M.G.d.T., C.C., L.M., C.S., G.M., K.B., M.A.B., F.M., E.D., and M.M. CORRESPONDING AUTHOR Correspondence to Michele Mondini. ETHICS DECLARATIONS COMPETING INTERESTS P.B., L.S., W.L.,
M.G.D.T., C.C., L.M., C.S., G.M., E.D. and M.M. declare grants from Roche Genentech, AstraZeneca, Merck Serono, Bristol-Myers Squibb, Boehringer Ingelheim, Eli Lilly and MSD, outside the
submitted work. E.D. declares consulting fees from Graegis. The remaining authors declare no competing interests. PEER REVIEW PEER REVIEW INFORMATION _Nature Communications_ thanks Udo
Gaipl, Etienne Meylan, Yuqiu Yang and the other, anonymous, reviewer(s) for their contribution to the peer review of this work. A peer review file is available. ADDITIONAL INFORMATION
PUBLISHER’S NOTE Springer Nature remains neutral with regard to jurisdictional claims in published maps and institutional affiliations. SUPPLEMENTARY INFORMATION SUPPLEMENTARY INFORMATION
PEER REVIEW FILE REPORTING SUMMARY SOURCE DATA SOURCE DATA RIGHTS AND PERMISSIONS OPEN ACCESS This article is licensed under a Creative Commons Attribution 4.0 International License, which
permits use, sharing, adaptation, distribution and reproduction in any medium or format, as long as you give appropriate credit to the original author(s) and the source, provide a link to
the Creative Commons licence, and indicate if changes were made. The images or other third party material in this article are included in the article’s Creative Commons licence, unless
indicated otherwise in a credit line to the material. If material is not included in the article’s Creative Commons licence and your intended use is not permitted by statutory regulation or
exceeds the permitted use, you will need to obtain permission directly from the copyright holder. To view a copy of this licence, visit http://creativecommons.org/licenses/by/4.0/. Reprints
and permissions ABOUT THIS ARTICLE CITE THIS ARTICLE Bergeron, P., Dos Santos, M., Sitterle, L. _et al._ Non-homogenous intratumor ionizing radiation doses synergize with PD1 and CXCR2
blockade. _Nat Commun_ 15, 8845 (2024). https://doi.org/10.1038/s41467-024-53015-9 Download citation * Received: 11 October 2023 * Accepted: 29 September 2024 * Published: 14 October 2024 *
DOI: https://doi.org/10.1038/s41467-024-53015-9 SHARE THIS ARTICLE Anyone you share the following link with will be able to read this content: Get shareable link Sorry, a shareable link is
not currently available for this article. Copy to clipboard Provided by the Springer Nature SharedIt content-sharing initiative