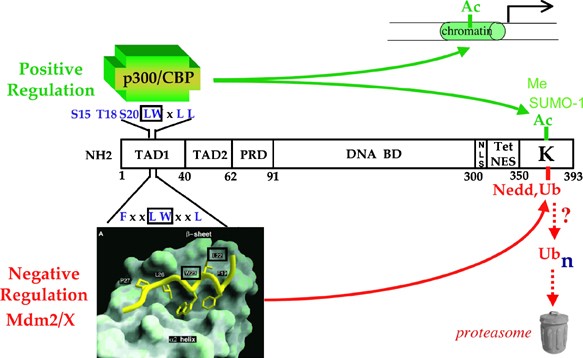
- Select a language for the TTS:
- UK English Female
- UK English Male
- US English Female
- US English Male
- Australian Female
- Australian Male
- Language selected: (auto detect) - EN
Play all audios:
ABSTRACT P53 is a transcription factor that can cause cells to be eliminated by apoptosis or senescent-like arrest upon its activation by irreparable genetic damage, excessively expressed
oncogenes, or a broad spectrum of other stresses. As P53 executes life and death decisions, its activity must be stringently regulated, which implies that it is not likely to be controlled
by a simple regulatory mechanism involving a binary on–off switch. This brief review will summarize a subset of the new information presented at the 10th P53 workshop in Dunedin, New Zealand
in November 2004 as well as very recent publications that provide new insights into the molecular regulators of P53. Data emerging from mouse models provide a fundamentally different view
of how P53 is regulated than suggested by more traditional _in vitro_ approaches. The differences between cell culture and mouse models demonstrate the importance of preserving
stoichiometric relationships between P53 and its various regulators to obtain an accurate view of the relevant molecular mechanisms that control P53 activity. SIMILAR CONTENT BEING VIEWED BY
OTHERS TISSUE SPECIFICITY AND SPATIO-TEMPORAL DYNAMICS OF THE P53 TRANSCRIPTIONAL PROGRAM Article 08 February 2023 _TP53_: THE UNLUCKIEST OF GENES? Article Open access 23 October 2024
SHIFTING THE PARADIGMS FOR TUMOR SUPPRESSION: LESSONS FROM THE P53 FIELD Article 08 June 2021 INTRODUCTION Twenty-five years ago, a 53–54 kDa cellular protein now referred to as P53 was
reported to interact with viral oncoproteins.1, 2, 3, 4, 5 Initial studies suggested that P53 functioned as an oncogenic protein. Interestingly, recent studies that modeled two such
mutations in the mouse suggested that they ‘gained function’ by binding to and attenuating the functions of the P53-related proteins P63 and P73.6 This provides one mechanism by which some
P53 mutants function as oncogenes. However, structural gene mutations that disable P53 function, or that could engender dominant-negative properties, have been reported in about 50% of human
cancers, including those that contribute most to morbidity and mortality (e.g., see http://www-p53.iarc.fr/index.html). Clear loss of both p53 alleles has been reported in a significant
fraction of human cancers.7 Together, these data demonstrate that p53 is an important tumor suppressor.8, 9 Importantly, a substantial fraction of human tumors express wild-type _p53_, but
its function is compromised by aberrant expression of proteins that negatively regulate it, such as Hdm2 and HdmX (Hdm2 or HdmX designate the human proteins, and Mdm2 and MdmX the mouse; the
corresponding genes are _hdm2_, _hdmx_, _mdm2_, _mdmx_).10, 11, 12, 13 (Below, for simplicity, these regulators are collectively referred to as Mdm2 or MdmX.) Together, the data show that
the P53 pathway is disabled in the majority, if not all, human cancers. P53 is an unstable transcription factor that can regulate numerous downstream targets to induce permanent or
reversible cell cycle arrest, apoptosis, and DNA repair.14, 15 While P53 has also been reported to induce apoptosis by nontranscriptional mechanisms,16, 17, 18 substantial evidence indicates
that its transcriptional functions are critical for effective tumor suppression.19 Signals elicited by stresses including DNA damage (e.g., see, see, Huang _et al._,20 Kastan _et al._,21
and Wahl and Carr22), short or abnormally structured telomeres,23, 24 high-level oncogene signaling,25, 26, 27, 28 hypoxia,29 and glucose availability30 activate P53. Cells that encounter
such conditions either arrest cell division or die if they possess a functional P53 pathway. By contrast, if P53 is dysfunctional, cells proliferate when exposed to oncogenic stimuli,
unrepaired DNA damage, or metabolic perturbations that induce chromosome instability.31, 32 Thus, by eliminating P53 function, key controls to prevent cell cycle entry under inappropriate,
potentially genome destabilizing conditions are lifted, allowing outgrowth of the genetically unstable variants that fuel tumor progression. Since P53 output has the potential to kill cells,
stringent regulatory mechanisms have evolved to prevent its errant activation, as well as to allow for rapid activation when appropriate. However, the regulatory mechanisms that enable the
P53 pathway to generate different transcriptional responses to different stimuli in different tissues remain to be defined. There is significant medical importance to having a clear idea of
P53 regulatory mechanisms as 3 million human cancers per year are estimated to contain a wild-type P53 protein whose function is attenuated. Understanding the mechanisms underlying such
negative regulation affords a substantial opportunity for therapeutic intervention. This possibility has been realized with the development of agents such as _cis_-imidazolines (e.g., Nutlin
3A) that interfere with Mdm2–P53 interactions to activate P53 in cells with overexpressed Mdm2.33 Below, I review data from cell culture and mouse models that provide a current view of
mechanisms that keep P53 off in unstressed cells, allow its activation in response to conditions that produce double-strand breaks, and then turn off P53 upon stress abatement. INTEGRATION
OF P53 STRUCTURE WITH REGULATION P53 structure suggests many potential levels at which its transcription function could be regulated (Figure 1). Transfection studies suggest that P53
contains one N-terminal transactivation domain (TAD1) comprising the first 40 amino acids,34 and a second (TAD2) consisting of amino acids 43–63 that is revealed upon inactivation of TAD1.35
A potential conformational switch may be contained within a domain comprising proline-X-X-proline motifs between the TAD and the large central DNA-binding domain.36, 37, 38, 39 A C-terminal
oligomerization domain is necessary to form tetramers.40 These tetramers not only constitute the most active transcriptional form of P5340, 41, 42, 43, 44, 45, 46, 47 but also conceal the
dominant nuclear export signal that lies within the oligomerization domain.48 The lysine-rich C-terminus constitutes a domain implicated in both positive regulation by post-translational
modifications involving acetylation,49, 50, 51, 52 methylation,53, 54 and sumoylation,55, 56 and negative regulation by ubiquitylation57 or neddylation.58 The C-terminus has also been
proposed to function as an allosteric regulator.59, 60, 61 However, recent data make it more likely that this domain enables P53 to bind nonspecifically to DNA to increase the rate at which
specific P53 response elements are detected through linear diffusion.62, 63 THE N-TERMINAL TAD Post-translational P53 modifications have been proposed to induce structural alterations to
stabilize the protein and to effect the chromatin modifications needed for transcriptional activation. The extreme N-terminal TAD has residues critical for the binding of both the
coactivators needed for transactivation, and the E3 ubiquitin ligase MDM2 that contributes most significantly to determining the abundance and short half-life of P53 in unstressed cells.19
Indeed, mutation of just two amino acids within this domain (L22Q, W23S in human, and L25Q, W26S in mouse; referred to as P53QS below) generates a stable but largely inactive P53 as the
mutant can neither interact with Mdm2 (and presumably MdmX, which has a similar P53-binding domain) nor with the required coactivators. One attractive model is that DNA damage activates the
ATM kinase to induce a phosphorylation cascade beginning with human ser15 (i.e., h-ser15), equivalent to mouse ser18 (m-ser18), which triggers the subsequent phosphorylation of h-thr18
(m-thr21) culminating in phosphorylation of h-ser20 (m-ser23).64 These residues constitute part of a helical domain that interacts with a hydrophobic pocket in the Mdm2 N-terminus.65 _In
vitro_ studies with peptides from this region indicate that thr18 phosphorylation can significantly destabilize this structure, leading to Mdm2 dissociation.66 Transfection studies suggest
that h-ser20 phosphorylation significantly destabilizes the Mdm2/P53 interaction,67 while others indicate that h-ser15 phosphorylation increases the affinity for p300/CBP.68, 69 These events
together have been suggested to stimulate P53 transactivation function. By contrast, other studies showed that P53 mutants in which all serine residues in the entire protein were changed to
alanine displayed wild-type stability and transactivation.19 Of great significance, while mouse mutants generated by homologous recombination to encode m-ser18ala appeared to exhibited
tissue-specific defects in p53 target gene activation, its capacity to suppress spontaneous tumor formation remained intact.70, 71, 72 Mice expressing the ser23ala mutation also exhibited
modest, tissue-specific deficiencies, but not the substantial destabilization and functional inactivation that would have been predicted were phosphorylation of this residue critical for
reducing Mdm2 binding.73, 74 These data are most consistent with N-terminal phosphorylations contributing to modulating tissue-specific responses rather than serving as an on–off switch as
occurs in _Drosophila_.75, 76 The interpretation of the significance of N-terminal phosphorylation on P53 function also depends on whether P53 contains two TADs. If it does, then mutations
in m-ser18 and m-thr21 might only inactivate the first one, but preserve the function of the second to allow some level of transactivation. The question of whether P53 contains more than one
functional TAD was raised at the Dunedin meeting. The human L22QW23S mutant was found by transfection analysis to retain some residual function, and residues 43–63 were subsequently
identified as a second potential TAD (see above). Recent data indicate that the second TAD mediates activation of the proapoptotic IGFBP3 gene, and that the basic C-terminus inhibits this
function.77 This interpretation is based on transfection studies, and it is important to emphasize that the function of the second TAD is revealed only in the context of P53 mutants deleted
for TAD1, which contains the Mdm2 binding site. Thus, TAD1 mutants should encode a very stable P53 variant capable of binding chromatin constitutively (see below). The idea that the
C-terminus is inhibitory to P53 function is inconsistent with other studies indicating that it mediates linear diffusion to augment the ability of P53 to find its response elements (see
below). Therefore, it remains to be determined whether the second putative TAD can contribute to P53 functions in the context of a structurally wild-type P53 with compromised function for
TAD1 while preserving stability control by Mdm2. A second approach to define the importance of transactivation for P53-mediated tumor suppression, and to assess the relative contribution of
each P53 TAD _in vivo_, uses homologous recombination to generate mice with inactivating mutations in TAD1. Mice expressing a _p53_ with the mouse equivalent of the L22QW23S mutations (i.e.,
L25QW26S, referred to as p53QS) that largely inactivate human TAD1 have been generated by three groups.78, 79, 80 Two previous studies showed that the p53QS mutant appeared to be completely
devoid of function.78, 79 However, we now know that the targeting construct we used to generate this mutant contained an additional alanine to valine mutation at codon 135 in the
DNA-binding domain (Ala135 to Val135). The Val135 mutation also generates a temperature-sensitive allele.81 Unfortunately, a second study did not attempt to produce mice expressing _p53QS_,
but did generate differentiated ES cells and reconstituted thymus expressing this allele.78 The data from both our studies and those of Chao _et al._,78 as well as our more recent
comparisons of the _p53QS_ alleles with Ala135 (p53QSA) or Val135 (p53QSV),81 show that the p53QS mutations themselves generate p53 molecules devoid of detectable transcriptional activity,
ability to induce cell cycle arrest or apoptosis, or suppress tumor formation under the conditions tested. In stark contrast to this conclusion, data presented at the Dunedin meeting showed
that an independently generated p53QS with Ala135 is embryonic lethal in heterozygotes.80 As no homozygous _p53QSA_ embryos were obtained, the source of the lethality remains to be
determined. It is possible that the P53QSA protein could stabilize and activate the remaining wild-type P53, that the mutant allele could retain transcription function in the proposed second
TAD, or that another mechanism such as that described below could underlie the observed lethality. Our recent studies provide another way of envisioning lethality generated by a stable, but
transcriptionally inert transcriptional regulator.81 We carefully compared the properties of endogenous p53QSV with exogenously expressed p53QSA (introduced into _p53_-null MEFs by
lentiviral infection, and clones expressing the same levels of P53QSV and P53QSA proteins were identified and analyzed). We detected no transactivation activity of either mutant on genes
such as _p21_, _mdm2_, and _Puma_, but, consistent with the results from the Attardi lab, we did detect bax transactivation by our _p53QSA_. However, _bax_ activation by either our p53QSV or
QSA MEFs or those from the Attardi lab was only twice that observed in _p53_-null cells. We have yet to observe induction of cell cycle arrest, apoptosis, or suppression of xenograft
formation by p53QSA or p53QSV.81 p53QSA and p53QSV are, as expected, extremely stable proteins (_T_1/2>8 h). There is, however, one very important difference between P53QSA and P53QSV
proteins. While p53QSA binds _p21_, _mdm2_, _Puma_, and _Noxa_ promoters constitutively and at levels equal to that of activated wild-type p53, p53QSV binds these promoters far less
efficiently. Interestingly, we found that the p53QSA generated in the Attardi lab is at least five times as abundant in MEFs as p53QSV in MEFs derived from the mice we generated, even though
both proteins are equivalently stable and p53 mRNA synthesis is similar for each allele.82 Based on all the available data, we propose the following explanations for the embryonic lethality
exhibited by the mice produced by Johnson _et al._80 First, the very high level of the p53QSA protein in their mice could exhibit low activity in particular cell types under specific
conditions _in vivo_ due to its constitutive chromatin binding and weak transactivation mediated by the second TAD. Another interpretation suggested by our recent data suggests a
transactivation-independent mechanism of lethality. That is, although p53QSA lacks measurable transactivation function, we speculate that its accumulation to high levels, combined with its
tight and constitutive binding to chromatin, could interfere with cellular transcription, replication, or other DNA-associated molecular events to engender lethality. THE C-TERMINUS AND P53
REGULATION The data summarized above lead us to suggest that there is one dominant TAD in the N-terminus, and that, as deduced from _in vitro_ studies, there are residues within it that are
essential for the control of both P53 stability and transactivation. A model that is accepted by most of the field has emerged in which the binding of coactivators competes for binding of
the negative regulators Mdm2 and MdmX to the N-terminus. This sets up a competition for competing modifications such as acetylation and ubiquitylation of key highly conserved lysines (six in
human, seven in mouse) in the extreme C-terminus.51 As described above, the C-terminus is likely to contribute to P53 function by increasing the efficiency with which it finds its specific
response elements. Indeed, elegant studies presented at Dunedin by C Prives showed that the C-terminus enables p53 to engage circular DNA molecules, regardless of whether they have a p53
response element. Deletion of the C-terminus reduces association with random DNA, as found earlier in studies of chromatin association.83 Once P53 engages random DNA, the rate at which it
detects specific P53 response elements is increased by linear diffusion.63 However, neither acetylation nor phosphorylation of the C-terminus affected linear diffusion or target gene
transactivation.63, 83 These _in vitro_ observations raise the question of whether C-terminal modifications affect P53 function _in vivo_. Human P53 mutants in which all six highly conserved
C-terminal lysine residues were mutated to arginine to prevent post-translational modifications including ubiquitylation and acetylation proved to be stable and more active than wild-type
P53.84, 85 However, it is difficult to recreate the normal stoichiometric relationships between P53, Mdm2, and MdmX using cotransfection of P53 with Mdm2 into human cancer cell lines that
contain other alterations that could affect p53 function. I presented data at the Dunedin meeting obtained from mice generated by homologous recombination to encode _p53_ with arginine
substituted for the seven C-terminal lysines that are analogous to the corresponding residues in human p53 (i.e., p537KR;86 also see87 for a study that generated a _p53_ allele in which six
lys were changed to arg but mice were not produced). We developed this model to determine the impact of C-terminal modifications on P53 regulation. While the _in vitro_ data suggested this
mutation should be lethal if homozygous, we observed normal Mendelian transmission and no evidence of early mortality, sex bias, or developmental abnormalities.86 Surprisingly, mouse P537KR
and wild-type P53 exhibit the same half-life, and like wild-type P53, p537KR is degraded by Mdm2-mediated proteasome-dependent proteolysis. _In vitro_ analyses showed that P537KR can be
ubiquitylated by Mdm2 after transfection, but the pattern was not the same as that of wild-type P53. As other studies indicate that lysines other than in the C-terminus may control P53
stability,88 it is formally possible that alternative lysine(s) are targeted for ubiquitylation when those in the C-terminus are not available. However, as P537KR has precisely the same
half-life as wild-type P53, it seems unlikely that Mdm2 could engage widely separated lysines with equal efficiency. Furthermore, while Mdm2 can polyubiquitylate itself, it can only
polyubiquitylate P53 at multiple lysines. This means that if P53 needs to be polyubiquitylated for degradation, this must require the action of an E4 ubiquitin ligase, such as p300.89 It is
difficult to reconcile the identical half-lives of these two proteins with the observation that Mdm2 is sufficient to meditate its own polyubiquitylation, while P53 requires both Mdm2 and an
E4. Thus, our data raise the intriguing possibility that P53 degradation may merely require association with Mdm2, and it is the self-ubiquitylation of Mdm2 that drives the degradation of
both proteins. However, this model seems simplistic as Mdm2 can also bind to the P53-related proteins P63 and P73, yet it does not mediate their degradation.90, 91 Consequently, there must
be other critical steps, such as correct presentation of the substrate to the proteasome, or engagement of linking molecules such as hHR23 to effect substrate degradation.92, 93 Clearly,
more research is needed to resolve the precise mechanism by which Mdm2 induces P53 degradation, and to reveal how Mdm2 selectively degrades p53. We also investigated the activity of P537KR
in cell culture and in thymocytes _in vivo._ P537KR and wild-type P53 proved to be experimentally indistinguishable in terms of their activation kinetics, ability to induce target genes,
cell cycle arrest, and apoptosis in MEFs. However, P537KR was activated at lower doses of ionizing radiation in mice when freshly explanted thymocytes were analyzed. Importantly, this
apparently increased activity of P537KR was also observed _in vitro_ when MEFs were grown according to a 3T3 passage protocol. We observed identical initial growth rates of MEFs expressing
wild type and P537KR, but MEFs encoding P537KR entered a senescent state from which immortal variants did not arise. This contrasts with MEFs encoding wild-type P53 that entered crisis, but
emerged as immortal variants. These data suggest that while the conserved C-terminal lysines and associated modifications are not essential for P53 control, they are likely to fine-tune P53
activity, and to ensure that the magnitude of a stress responses is appropriate. This fine-tuning is likely to be critical for optimal lifespan of metazoans to guarantee that cells that
undergo numerous divisions, or at risk of stress exposure, do not experience errant P53 activation. THE PROLINE-RICH DOMAIN (PRD) AND MDM2 INTERACTION P53 is exquisitely sensitive to Mdm2
expression levels. For example, mice expressing a hypomorphic _mdm2_ allele produced 30–50% of the normal Mdm2 protein level.94 This resulted in precocious P53 activation in lymphoid tissue
and in some epithelial cells. Recent studies showed that _mdm2_+/− heterozygous mice were far more resistant to the development of lymphoid tumors induced by expression of the _Eu-Myc_
transgene.95 Finally, earlier onset and increased cancer predisposition has been reported in premenopausal women in whom a single-nucleotide polymorphism changes a T to a G in the _mdm2_
promoter.96 This base change creates a binding site for the ubiquitous transcription factor Sp1 adjacent to a response element for the estrogen receptor, which apparently together contribute
to two- to four-fold elevated Mdm2 levels that attenuate P53 function. Together, these data clearly show that Mdm2 abundance is an important determinant of the output of the P53 stress
response pathway. One way to modulate the impact of Mdm2 on P53 function is to regulate the efficiency with which it binds P53. As stated above, N-terminal phosphorylation may participate in
this, but its importance appears more significant in specific cell types. Another region that may affect Mdm2 binding consists of a loosely conserved PRD between the TAD and the DNA-binding
domain. This region contains a variable number of PXXP motifs, where P=proline and X=any amino acid. PXXP motifs provide potential interaction sites with proteins containing src homology 3
motifs, and the PXXP domains of P53 have been reported to interact with proteins including Mdm2,38, 39, 97 p300,98 WWOX1,99 and the corepressor mSin3a.100 Early studies showed that deletion
of the PRD in human P53 alter P53 transactivation in such a way as to preserve the apoptotic function, while compromising cell cycle arrest.101 Initial studies suggested that PRD deletion
reduced the affinity of P53 for apoptotic target genes,102, 103 but analyses performed at lower, more natural expression levels, revealed reduced regulation of a broader spectrum of target
genes.104 Recent analyses have provided a potential molecular mechanism for the compromised functionality of P53 lacking the PRD. DNA damage can induce phosphorylation of multiple threonine
residues in threonine–proline motifs. This creates sites that can be bound by the prolyl isomerase Pin1, which can then induce _cis_–_trans_ isomerization of the adjacent proline. Prolyl
isomerization has been reported to reduce Mdm2 binding, and to stabilize and activate P53.36, 37, 39 Deleting the prolines, or mutating the threonine to nonphosphorylatable alanine, prevents
the required prolyl isomerization, and P53 damage responses appear to be compromised in Pin1-deficient MEFs. A recent twist on this story was added by Ygal Haupt at the Dunedin meeting,
when he reported that phosphorylation of thr81 is required to recruit Pin1, and that Pin1 recruitment is required for efficient association with the damage-activated kinase Chk2.39 Chk2
recruitment induces phosphorylation of ser20, which then reduces affinity for Mdm2, allowing P53 activation. Importantly, some humans with the Li–Fraumeni cancer predisposition syndrome have
been reported to have chk2 mutations,105, 106 while others encode mutant _p53_ with proline 82 replaced by leucine (i.e., pro82leu).107 The latter data suggest the importance of pro82 as an
important contributor to P53 function _in vivo._ Consistent with this interpretation, Haupt described transfection studies showing that P53 Pro82Leu accumulated less efficiently after
ionizing radiation, and exhibited reduced ability to activate a p21 promoter-driven luciferase reporter.39 These data suggest that the PRD may be important for regulating P53 output by
fine-tuning Mdm2 binding. One note of caution: earlier studies by Dumaz and Meek showed that the impact of deleting the PRD on P53 activation was critically dependent on the ratio of Mdm2 to
P53.97 Therefore, firm assessment of the contribution of the PRD to P53 control will await the generation and analysis of mice expressing _p53_ with a PRD deletion, and others in which the
prolines and threonines have been mutated to rigorously evaluate the validity of the conformational hypothesis advanced above. NEW THOUGHTS ON AN OLD DOGMA Over 36 000 papers addressing p53
function have been published since its discovery 25 years ago. By contrast, a fraction of this number has dealt with Mdm2 and MdmX, which partly reflects their more recent discoveries.19,
57, 108, 109 However, Mdm2 and MdmX are clearly critical negative regulators of P53, and their functions are nonoverlapping since deletion of either engenders early embryonic lethality.110,
111, 112, 113, 114 This raises the possibility that important aspects of P53 control remain to be elucidated due to the paucity of attention focused on Mdm2 and MdmX and proteins that
control their functionality, such as Arf,115, 116 gankyrin,117, 118 and the ubiquitin-specific protease HAUSP (herpes virus-associated ubiquitin-specific protease) (Li _et al._,119, 120 and
Meulmeester _et al._121; and see below). Another puzzle is why this system requires the two similar RING domain proteins Mdm2 and MdmX to each serve as a P53-negative regulator. Below, I
present a model I proposed at the Dunedin meeting in which I divided p53 regulation into four phases, and accounts for the kinetic relationships between p53, Mdm2, and MdmX we had observed
at that time. I will review recent unpublished and published data relevant to this model (Figure 2). PHASE 1: HOMEOSTASIS IN UNSTRESSED CELLS P53 is maintained at low levels and in an
inactive state in unstressed cells. This can be achieved in several ways. First, Mdm2 can bind to P53 to mediate proteasomal degradation.122, 123 Second, Mdm2 can bind to the P53 TAD to
prevent recruitment of coactivators that access the same site.124 Third, as described by Moshe Oren125 in Dunedin, Mdm2 can monoubiquitinate the histones located in the vicinity of the P53
response element when a P53–Mdm2 complex engages chromatin, and Mdm2 can also monoubiquitinate histones in a P53-independent manner when it is overexpressed. Less appreciated is the
contribution of MdmX to attenuating P53 function in unstressed cells. This could be substantial as MdmX is produced constitutively and is very stable (see Marine and Jochemsen108, 109 for
reviews). The molecular abundance of Mdm2 relative to MdmX in nonstressed and stressed cells remains to be determined. Thus, P53 is held at bay in unstressed cells by interactions with the
two negative regulators, Mdm2 and MdmX. What then controls the levels of Mdm2 and MdmX? The Mdm2 level in unstressed cells is determined by a number of factors including, but not limited to
the following: (1) P53-dependent transactivation of the Mdm2 gene (see Lahav _et al._125 for recent kinetic modeling); (2) mitogen-dependent activation of factors such as Erk that also
transactivate Mdm2;126 (3) mitogen-dependent post-translational modifications that modulate Mdm2 stability (e.g., see Ashcroft _et al._127 and Gottlieb _et al._128); and (4) interaction with
HAUSP.119, 120, 121, 129 The factors that regulate MdmX abundance have not been widely studied, but one that appears increasingly important involves interaction with HAUSP.121 HAUSP was
first identified to participate in P53 control by Gu and co-workers as a P53-associated protein.120 Inactivating HAUSP by siRNA or by homologous recombination in HCT116 cells resulted in
Mdm2 and MdmX destabilization.120, 121, 130 The importance of HAUSP in regulating MdmX level is demonstrated by the lack of detectable MdmX in HAUSP-deficient cells.121 As eliminating HAUSP
greatly destabilized both Mdm2 and MdmX, P53 became stabilized and activated.130 These observations imply that HAUSP interaction with, and deubiquitination of, Mdm2 and MdmX contributes
significantly to their steady-state levels in unstressed cells. As MdmX has a longer half-life than either Mdm2 or P53, it is reasonable to speculate that MdmX may be the preferred substrate
for HAUSP-mediated deubiquitination. To summarize regulation of Phase 1: Mdm2 accumulates as a consequence of transcriptional activation by P53 and mitogen-activated factors, as well as by
‘stabilization’ through HAUSP interaction. While Mdm2 can ubiquitinate MdmX, MdmX is deubiquitinated and stabilized via interaction with HAUSP. The aggregate levels of Mdm2 and MdmX
determine both P53 abundance and transcriptional activity. Mdm2-mediated ubiquitination of histones may provide an additional means of controlling P53 transcription functions. PHASE 2: EARLY
ACTIVATION DNA damage leads to the activation of ATM and other kinases within minutes, as well as phosphorylation of N-terminal serines that may modulate association with Mdm2/X and
coactivators. However, of equal importance, these kinases also rapidly phosphorylate Mdm2 and MdmX.131, 132, 133, 134 We showed that phosphorylation of MDM2 by damage kinases mediates its
accelerated degradation early in the damage response.135 Studies from the Yuan and Chen labs showed that DNA damage also leads to MdmX degradation,132, 136 and we now know this requires the
RING and acidic domains and ubiquitin ligase activity of Mdm2.131, 137, 138, 139 A picture of what triggers the accelerated degradation of Mdm2 early in the damage response, and what
converts MdmX from a stable to an unstable protein is now emerging. Recent work from the Shiloh and Jochemsen labs show that the damage-induced phosphorylations on Mdm2 and MdmX destabilize
both proteins by preventing them from interacting with HAUSP.121, 134 Another study provided a somewhat different explanation that phosphorylation increased association between Mdm2 and
MdmX.140 Regardless of which mechanism is correct, the data taken together imply that DNA damage triggers accelerated degradation of both negative regulators. However, early in the DNA
damage response in normal fibroblasts and epithelial cells, it appears that MdmX levels do not decline immediately, and that P53 target genes are activated weakly. Indeed, full activation
takes between 1 and 2 h.135 The next section provides one explanation of this delay. PHASE 3. FULL ACTIVATION It is clear that full P53 activation occurs only after a lag of about 2 h.135
Damage kinases are active over this time, P53 remains phosphorylated at h-ser15, and presumably Mdm2 and Mdmx are also phosphorylated over this interval. One of the first genes activated by
P53 is Mdm2, but early in the damage response, the Mdm2 that is made is unstable, likely because it is phosphorylated and cannot interact with HAUSP. Mdm2 does accumulate over time, however,
and this correlates with progressively decreasing MdmX levels. This raises the possibility that P53 activates Mdm2 so that its increased abundance ensures MdmX degradation. This model
predicts that conditions that lead to P53 activation in the absence of activation of damage kinases should also lead to MdmX degradation due to the elevated Mdm2 abundance. As shown by Lubo
Vassilev at the Dunedin meeting, P53 can be fully activated by Nutlin3a, which prevents Mdm2 from binding to P53.141 Indeed, we found that Nutlin3a results in substantial increases in Mdm2
abundance, and that MdmX levels decline in parallel (J Stommel, M Wade, M Tang, and G Wahl, unpublished data). Importantly, we also showed that proteasome inhibitors prevent P53 activation
after DNA damage, even though P53 was phosphorylated in its N-terminus.135 By contrast, Nutlin addition during proteasome inhibitor treatment enabled P53 activation by reducing Mdm2 (and
presumably)–MdmX interaction with P53.135 Together, the data indicate that P53 activation requires Mdm2 and Mdmx phosphorylation to decrease HAUSP interaction with both proteins, the net
effect of which is to increase Mdm2-mediated self-ubiquitylation and ubiquitylation of MdmX. This decreases the levels of Mdm2 at early time points and as a consequence activates P53,
resulting in increased Mdm2 transactivation. The increased levels of Hdm2 can then degrade MdmX, resulting in full activation of P53. In this model, P53 activation requires a _positive
feedback loop_ in which increasing Mdm2 abundance titrates the amount of MdmX degradation to assure the fine-tuning of the timing and magnitude of the P53 transcriptional response. PHASE 4:
ATTENUATION The components of this system also provide the potential to attenuate P53 signaling should a stress dissipate or DNA damage be repaired. I suggest this could occur in the
following way. ATM and P53 ser15 phosphorylation return to background levels within 4–6 h of induction of DNA damage with a low dose of the radiomimetic agent neocarzinostatin.135 In normal
human fibroblasts, Mdm2 levels are high at 4 h, and start to decline thereafter, along with a parallel decrease in P53 transactivation. In light of the data summarized above, it is
reasonable to propose that the damage-phosphorylated Mdm2 pool is replaced with a nonphosphorylated pool as a consequence of new synthesis and diminished abundance of activated ATM. The
elevated levels of Mdm2 should be able to interact with and inactivate P53 by the mechanisms described above. Furthermore, in the absence of activated damage kinases, MdmX will be allowed to
interact with HAUSP, leading to deubiquitination and stabilization. This provides a second barrier to continued P53 activation. PERSPECTIVES The integration of recent data described above
provides a new way of conceptualizing P53 regulation. Homeostasis is maintained by the well-known negative feedback loop. A new twist to the loop is provided by the idea that the activation
of Mdm2 during a stress response actually creates a positive feedback loop in which Mdm2 mediates MdmX degradation to achieve full P53 activation. This elegant system enables gradual and
tightly controlled increases in P53 activity rather than an all-or-none response that might be created were the system solely controlled solely by phosphorylation. Finally, the reservoir of
Mdm2 created by P53-mediated activation enables attenuation of the P53 response since most of the MdmX is eliminated in the activation phase. This model accounts for most of the major
regulators known to operate in this system, and it is consistent with the kinetics of the response in the normal fibroblasts and epithelial cells we have analyzed. It is clear that the
system is very sensitive to small changes in the stoichiometric relationships between HAUSP, Mdm2, MdmX, and P53, and it is likely that proteins that regulate Mdm2 function, such as Arf or
Gankyrin, may enable finer tuning of the system, or be required for responses to stressors other than DNA damage. Clearly, an important goal for the near future will be to determine the
precise numbers of each of these molecules in normal and neoplastic cells, and to determine how differences in stoichiometry affect the dynamics and magnitude of stress-activated responses.
We have begun a cursory analysis of cell lines with wild-type P53, and have found substantial variations in the relative abundance of each molecule. This may well explain the attenuation of
the P53 response in the numerous cancers expressing wild-type P53. An important unresolved question concerns the molecular mechanism that switches Mdm2 target specificity from P53 to MdmX.
Clearly, one component of the mechanism is determined by whether HAUSP interacts with and deubiquitinates Mdm2 and MdmX. However, as described above, the binding of Mdm2 to P53 may be all
that is needed for P53 to be degraded. As N-terminal phosphorylation of P53 seems unlikely to prevent Mdm2 from binding to P53, we need to determine whether P53 stabilization involves
preventing Mdm2 from interacting with P53, or whether additional factors are involved. As one example, perhaps proteins that connect Mdm2 to the proteasome, such as hHR23, comprise part of
the switch.92, 93 The excitement in this field has grown with the successful isolation of a variety of compounds such as the Nutlins that can activate wild-type P53 in some tumor lines. What
we now need to determine is whether the different expression patterns of the key proteins that control P53 function will impact on the utility of these agents, or if the molecular
signatures of cancer cells can be used to define subsets of patients most likely to respond to P53 activator therapies. Of significance, as MdmX has emerged to play an increasingly important
role in P53 activation, the optimal drugs for p53 activation clearly need to target both Mdm2 and MdmX to prevent both from interacting with and inhibiting P53. ABBREVIATIONS * TAD1:
transactivation domain REFERENCES * Kress M, May E, Cassingena R and May P (1979) Simian virus 40-transformed cells express new species of proteins precipitable by anti-Simian virus 40 tumor
serum. _J. Virol._ 31: 472–483. CAS PubMed PubMed Central Google Scholar * Melero JA, Stitt DT, Mangel WF and Carroll RB (1979) Identification of new polypeptide species (48–55K)
immunoprecipitable by antiserum to purified large T antigen and present in SV40-infected and -transformed cells. _Virology_ 93: 466–480. CAS PubMed Google Scholar * DeLeo AB, Jay G,
Appella E, Dubois GC, Law LW and Old LJ (1979) Detection of a transformation-related antigen in chemically induced sarcomas and other transformed cells of the mouse. _Proc. Natl. Acad. Sci.
USA_ 76: 2420–2424. CAS PubMed PubMed Central Google Scholar * Lane DP and Crawford LV (1979) T antigen is bound to a host protein in SV40-transformed cells. _Nature_ 278: 261–263. CAS
PubMed Google Scholar * Linzer DI and Levine AJ (1979) Characterization of a 54K Dalton cellular SV40 tumor antigen present in SV40-transformed cells and uninfected embryonal carcinoma
cells. _Cell_ 17: 43–52. CAS PubMed Google Scholar * Olive KP, Tuveson DA, Ruhe ZC, Yin B, Willis NA, Bronson RT, Crowley D and Jacks T (2004) Mutant p53 gain of function in two mouse
models of Li–Fraumeni syndrome. _Cell_ 119: 847–860. CAS PubMed Google Scholar * Hollstein M, Sidransky D, Vogelstein B and Harris CC (1991) p53 mutations in human cancers. _Science_ 253:
49–53. CAS PubMed Google Scholar * Malkin D, Li FP, Strong LC, Fraumeni Jr JF, Nelson CE, Kim DH, Kassel J, Gryka MA, Bischoff FZ, Tainsky MA and Friend SH (1990) Germ line p53 mutations
in a familial syndrome of breast cancer, sarcomas, and other neoplasms. _Science_ 250: 1233–1238. CAS PubMed Google Scholar * Srivastava S, Zou ZQ, Pirollo K, Blattner W and Chang EH
(1990) Germ-line transmission of a mutated p53 gene in a cancer-prone family with Li–Fraumeni syndrome. _Nature_ 348: 747–749. CAS PubMed Google Scholar * Danovi D, Meulmeester E, Pasini
D, Migliorini D, Capra M, Frenk R, de Graaf P, Francoz S, Gasparini P, Gobbi A, Helin K, Pelicci PG, Jochemsen AG and Marine JC (2004) Amplification of Mdmx (or Mdm4) directly contributes to
tumor formation by inhibiting p53 tumor suppressor activity. _Mol. Cell. Biol._ 24: 5835–5843. CAS PubMed PubMed Central Google Scholar * Momand J, Jung D, Wilczynski S and Niland J
(1998) The MDM2 gene amplification database. _Nucleic Acids Res._ 26: 3453–3459. CAS PubMed PubMed Central Google Scholar * Reifenberger G, Liu L, Ichimura K, Schmidt EE and Collins VP
(1993) Amplification and overexpression of the MDM2 gene in a subset of human malignant gliomas without p53 mutations. _Cancer Res._ 53: 2736–2739. CAS PubMed Google Scholar *
Riemenschneider MJ, Buschges R, Wolter M, Reifenberger J, Bostrom J, Kraus JA, Schlegel U and Reifenberger G (1999) Amplification and overexpression of the MDM4 (MDMX) gene from 1q32 in a
subset of malignant gliomas without TP53 mutation or MDM2 amplification. _Cancer Res._ 59: 6091–6096. CAS PubMed Google Scholar * Hoh J, Jin S, Parrado T, Edington J, Levine AJ and Ott J
(2002) The p53MH algorithm and its application in detecting p53-responsive genes. _Proc. Natl. Acad. Sci. USA_ 99: 8467–8472. CAS PubMed PubMed Central Google Scholar * Vogelstein B,
Lane D and Levine AJ (2000) Surfing the p53 network. _Nature_ 408: 307–310. CAS PubMed Google Scholar * Caelles C, Helmberg A and Karin M (1994) p53-dependent apoptosis in the absence of
transcriptional activation of p53-target genes. _Nature_ 370: 220–223. CAS PubMed Google Scholar * Chipuk JE, Kuwana T, Bouchier-Hayes L, Droin NM, Newmeyer DD, Schuler M and Green DR
(2004) Direct activation of Bax by p53 mediates mitochondrial membrane permeabilization and apoptosis. _Science_ 303: 1010–1014. CAS PubMed Google Scholar * Marchenko ND, Zaika A and Moll
UM (2000) Death signal-induced localization of p53 protein to mitochondria. A potential role in apoptotic signaling. _J. Biol. Chem._ 275: 16202–16212. CAS PubMed Google Scholar * Wahl
GM, Stommel JM, Krummel KA and Wade M (2005) Gatekeepers of the guardian: p53 regulation by post-translational modification, mdm2 and mdmx. In _25 Years of p53 Research_, Wiman K, Hainaut P
(eds) (Netherlands: Springer) pp. 73–113. Google Scholar * Huang L-C, Clarkin KC and Wahl GM (1996) Sensitivity and selectivity of the p53-mediated DNA damage sensor. _Proc. Natl. Acad.
Sci. USA_ 93: 4827–4832. CAS PubMed PubMed Central Google Scholar * Kastan MB, Onyekwere O, Sidransky D, Vogelstein B and Craig RW (1991) Participation of p53 protein in the cellular
response to DNA damage. _Cancer Res._ 51 (Part 1): 6304–6311. CAS PubMed Google Scholar * Wahl GM and Carr AM (2001) The evolution of diverse biological responses to DNA damage: insights
from yeast and p53. _Nat. Cell Biol._ 3: E277–E286. CAS PubMed Google Scholar * Chin L, Artandi SE, Shen Q, Tam A, Lee SL, Gottlieb GJ, Greider CW and DePinho RA (1999) p53 deficiency
rescues the adverse effects of telomere loss and cooperates with telomere dysfunction to accelerate carcinogenesis. _Cell_ 97: 527–538. CAS PubMed Google Scholar * Karlseder J, Broccoli
D, Dai Y, Hardy S and de Lange T (1999) p53- and ATM-dependent apoptosis induced by telomeres lacking TRF2. _Science_ 283: 1321–1325. CAS PubMed Google Scholar * Denko NC, Giaccia AJ,
Stringer JR and Stambrook PJ (1994) The human Ha-ras oncogene induces genomic instability in murine fibroblasts within one cell cycle. _Proc. Natl. Acad. Sci. USA_ 91: 5124–5128. CAS PubMed
PubMed Central Google Scholar * Felsher DW and Bishop JM (1999) Transient excess of MYC activity can elicit genomic instability and tumorigenesis. _Proc. Natl. Acad. Sci. USA_ 96:
3940–3944. CAS PubMed PubMed Central Google Scholar * Sherr CJ (2001) The INK4a/ARF network in tumour suppression. _Nat. Rev. Mol. Cell. Biol._ 2: 731–737. CAS PubMed Google Scholar *
Vafa O, Wade M, Kern S, Beeche M, Pandita TK, Hampton GM and Wahl GM (2002) c-Myc can induce DNA damage, increase reactive oxygen species, and mitigate p53 function: a mechanism for
oncogene-induced genetic instability. _Mol. Cell_ 9: 1031–1044. CAS PubMed Google Scholar * Alarcon R, Koumenis C, Geyer RK, Maki CG and Giaccia AJ (1999) Hypoxia induces p53 accumulation
through MDM2 down-regulation and inhibition of E6-mediated degradation. _Cancer Res._ 59: 6046–6051. CAS PubMed Google Scholar * Jones RG, Plas DR, Kubek S, Buzzai M, Mu J, Xu Y,
Birnbaum MJ and Thompson CB (2005) AMP-activated protein kinase induces a p53-dependent metabolic checkpoint. _Mol. Cell_ 18: 283–293. CAS PubMed Google Scholar * Livingstone LR, White A,
Sprouse J, Livanos E, Jacks T and Tlsty TD (1992) Altered cell cycle arrest and gene amplification potential accompany loss of wild-type p53. _Cell_ 70: 923–935. CAS PubMed Google Scholar
* Yin Y, Tainsky MA, Bischoff FZ, Strong LC and Wahl GM (1992) Wild-type p53 restores cell cycle control and inhibits gene amplification in cells with mutant p53 alleles. _Cell_ 70:
937–948. CAS PubMed Google Scholar * Vassilev LT, Vu BT, Graves B, Carvajal D, Podlaski F, Filipovic Z, Kong N, Kammlott U, Lukacs C, Klein C, Fotouhi N and Liu EA (2004) _In vivo_
activation of the p53 pathway by small-molecule antagonists of MDM2. _Science_ 303: 844–848. CAS PubMed Google Scholar * Lin J, Chen J, Elenbaas B and Levine AJ (1994) Several hydrophobic
amino acids in the p53 amino-terminal domain are required for transcriptional activation, binding to mdm-2 and the adenovirus 5 E1B 55-kD protein. _Genes Dev._ 8: 1235–1246. CAS PubMed
Google Scholar * Zhu J, Zhou W, Jiang J and Chen X (1998) Identification of a novel p53 functional domain that is necessary for mediating apoptosis. _J. Biol. Chem._ 273: 13030–13036. CAS
PubMed Google Scholar * Zacchi P, Gostissa M, Uchida T, Salvagno C, Avolio F, Volinia S, Ronai Z, Blandino G, Schneider C and Del Sal G (2002) The prolyl isomerase Pin1 reveals a mechanism
to control p53 functions after genotoxic insults. _Nature_ 419: 853–857. CAS PubMed Google Scholar * Zheng H, You H, Zhou XZ, Murray SA, Uchida T, Wulf G, Gu L, Tang X, Lu KP and Xiao ZX
(2002) The prolyl isomerase Pin1 is a regulator of p53 in genotoxic response. _Nature_ 419: 849–853. CAS PubMed Google Scholar * Berger M, Vogt Sionov R, Levine AJ and Haupt Y (2001) A
role for the polyproline domain of p53 in its regulation by Mdm2. _J. Biol. Chem._ 276: 3785–3790. CAS PubMed Google Scholar * Berger M, Stahl N, Del Sal G and Haupt Y (2005) Mutations in
proline 82 of p53 impair its activation by Pin1 and Chk2 in response to DNA damage. _Mol. Cell. Biol._ 25: 5380–5388. CAS PubMed PubMed Central Google Scholar * Jeffrey PD, Gorina S and
Pavletich NP (1995) Crystal structure of the tetramerization domain of the p53 tumor suppressor at 1.7 angstroms. _Science_ 267: 1498–1502. CAS PubMed Google Scholar * Arrowsmith CH and
Morin P (1996) New insights into p53 function from structural studies. _Oncogene_ 12: 1379–1385. CAS PubMed Google Scholar * Clore GM, Ernst J, Clubb R, Omichinski JG, Kennedy WM,
Sakaguchi K, Appella E and Gronenborn AM (1995) Refined solution structure of the oligomerization domain of the tumour suppressor p53. _Nat. Struct. Biol._ 2: 321–333. CAS PubMed Google
Scholar * Miller M, Lubkowski J, Rao JK, Danishefsky AT, Omichinski JG, Sakaguchi K, Sakamoto H, Appella E, Gronenborn AM and Clore GM (1996) The oligomerization domain of p53: crystal
structure of the trigonal form. _FEBS Lett._ 399: 166–170. CAS PubMed Google Scholar * Maki CG (1999) Oligomerization is required for p53 to be efficiently ubiquitinated by MDM2. _J.
Biol. Chem._ 274: 16531–16535. CAS PubMed Google Scholar * Clore GM, Omichinski JG, Sakaguchi K, Zambrano N, Sakamoto H, Appella E and Gronenborn AM (1994) High-resolution structure of
the oligomerization domain of p53 by multidimensional NMR [see comments] [published erratum appears in _Science_ 1995 Mar 10;267(5203):1515]. _Science_ 265: 386–391. CAS PubMed Google
Scholar * Wang P, Reed M, Wang Y, Mayr G, Stenger JE, Anderson ME, Schwedes JF and Tegtmeyer P (1994) p53 domains: structure, oligomerization, and transformation. _Mol. Cell. Biol._ 14:
5182–5191. CAS PubMed PubMed Central Google Scholar * McLure KG and Lee PW (1998) How p53 binds DNA as a tetramer. _EMBO J._ 17: 3342–3350. CAS PubMed PubMed Central Google Scholar *
Stommel JM, Marchenko ND, Jimenez GS, Moll UM, Hope TJ and Wahl GM (1999) A leucine-rich nuclear export signal in the p53 tetramerization domain: regulation of subcellular localization and
p53 activity by NES masking. _EMBO J._ 18: 1660–1672. CAS PubMed PubMed Central Google Scholar * Gu W and Roeder RG (1997) Activation of p53 sequence-specific DNA binding by acetylation
of the p53 C-terminal domain. _Cell_ 90: 595–606. CAS PubMed Google Scholar * Gu W, Shi XL and Roeder RG (1997) Synergistic activation of transcription by CBP and p53. _Nature_ 387:
819–823. CAS PubMed Google Scholar * Li M, Luo J, Brooks CL and Gu W (2002) Acetylation of p53 inhibits its ubiquitination by Mdm2. _J. Biol. Chem._ 277: 50607–50611. CAS PubMed Google
Scholar * Prives C and Manley JL (2001) Why is p53 acetylated? _Cell_ 107: 815–818. CAS PubMed Google Scholar * An W, Kim J and Roeder RG (2004) Ordered cooperative functions of PRMT1,
p300, and CARM1 in transcriptional activation by p53. _Cell_ 117: 735–748. CAS PubMed Google Scholar * Chuikov S, Kurash JK, Wilson JR, Xiao B, Justin N, Ivanov GS, McKinney K, Tempst P,
Prives C, Gamblin SJ, Barlev NA and Reinberg D (2004) Regulation of p53 activity through lysine methylation. _Nature_ 432: 353–360. CAS PubMed Google Scholar * Gostissa M, Hengstermann A,
Fogal V, Sandy P, Schwarz SE, Scheffner M and Del Sal G (1999) Activation of p53 by conjugation to the ubiquitin-like protein SUMO-1. _EMBO J._ 18: 6462–6471. CAS PubMed PubMed Central
Google Scholar * Rodriguez MS, Desterro JM, Lain S, Midgley CA, Lane DP and Hay RT (1999) SUMO-1 modification activates the transcriptional response of p53. _EMBO J._ 18: 6455–6461. CAS
PubMed PubMed Central Google Scholar * Michael D and Oren M (2003) The p53–Mdm2 module and the ubiquitin system. _Semin. Cancer Biol._ 13: 49–58. CAS PubMed Google Scholar * Xirodimas
DP, Saville MK, Bourdon JC, Hay RT and Lane DP (2004) Mdm2-mediated NEDD8 conjugation of p53 inhibits its transcriptional activity. _Cell_ 118: 83–97. CAS PubMed Google Scholar * Hupp TR
and Lane DP (1994) Allosteric activation of latent p53 tetramers. _Curr. Biol._ 4: 865–875. CAS PubMed Google Scholar * Hupp TR, Meek DW, Midgley CA and Lane DP (1993) Activation of the
cryptic DNA binding function of mutant forms of p53. _Nucleic Acids Res._ 21: 3167–3174. CAS PubMed PubMed Central Google Scholar * Waterman JL, Shenk JL and Halazonetis TD (1995) The
dihedral symmetry of the p53 tetramerization domain mandates a conformational switch upon DNA binding. _EMBO J._ 14: 512–519. CAS PubMed PubMed Central Google Scholar * Ahn J and Prives
C (2001) The C-terminus of p53: the more you learn the less you know. _Nat. Struct. Biol._ 8: 730–732. CAS PubMed Google Scholar * McKinney K, Mattia M, Gottifredi V and Prives C (2004)
p53 linear diffusion along DNA requires its C terminus. _Mol. Cell_ 16: 413–424. CAS PubMed Google Scholar * Sakaguchi K, Saito S, Higashimoto Y, Roy S, Anderson CW and Appella E (2000)
Damage-mediated phosphorylation of human p53 threonine 18 through a cascade mediated by a casein 1-like kinase. Effect on Mdm2 binding. _J. Biol. Chem._ 275: 9278–9283. CAS PubMed Google
Scholar * Kussie PH, Gorina S, Marechal V, Elenbaas B, Moreau J, Levine AJ and Pavletich NP (1996) Structure of the MDM2 oncoprotein bound to the p53 tumor suppressor transactivation
domain. _Science_ 274: 948–953. CAS PubMed Google Scholar * Schon O, Friedler A, Bycroft M, Freund SM and Fersht AR (2002) Molecular mechanism of the interaction between MDM2 and p53. _J.
Mol. Biol._ 323: 491–501. CAS PubMed Google Scholar * Unger T, Juven-Gershon T, Moallem E, Berger M, Vogt Sionov R, Lozano G, Oren M and Haupt Y (1999) Critical role for Ser20 of human
p53 in the negative regulation of p53 by Mdm2. _EMBO J._ 18: 1805–1814. CAS PubMed PubMed Central Google Scholar * Dumaz N and Meek DW (1999) Serine15 phosphorylation stimulates p53
transactivation but does not directly influence interaction with HDM2. _EMBO J._ 18: 7002–7010. CAS PubMed PubMed Central Google Scholar * Lambert PF, Kashanchi F, Radonovich MF,
Shiekhattar R and Brady JN (1998) Phosphorylation of p53 serine 15 increases interaction with CBP. _J. Biol. Chem._ 273: 33048–33053. CAS PubMed Google Scholar * Borges HL, Chao C, Xu Y,
Linden R and Wang JY (2004) Radiation-induced apoptosis in developing mouse retina exhibits dose-dependent requirement for ATM phosphorylation of p53. _Cell Death Differ._ 11: 494–502. CAS
PubMed Google Scholar * Chao C, Hergenhahn M, Kaeser MD, Wu Z, Saito S, Iggo R, Hollstein M, Appella E and Xu Y (2003) Cell type- and promoter-specific roles of Ser18 phosphorylation in
regulating p53 responses. _J. Biol. Chem._ 278: 41028–41033. CAS PubMed Google Scholar * Sluss HK, Armata H, Gallant J and Jones SN (2004) Phosphorylation of serine 18 regulates distinct
p53 functions in mice. _Mol. Cell. Biol._ 24: 976–984. CAS PubMed PubMed Central Google Scholar * MacPherson D, Kim J, Kim T, Rhee BK, Van Oostrom CT, DiTullio RA, Venere M, Halazonetis
TD, Bronson R, De Vries A, Fleming M and Jacks T (2004) Defective apoptosis and B-cell lymphomas in mice with p53 point mutation at Ser 23. _EMBO J._ 23: 3689–3699. CAS PubMed PubMed
Central Google Scholar * Wu Z, Earle J, Saito S, Anderson CW, Appella E and Xu Y (2002) Mutation of mouse p53 Ser23 and the response to DNA damage. _Mol. Cell. Biol._ 22: 2441–2449. CAS
PubMed PubMed Central Google Scholar * Brodsky MH, Weinert BT, Tsang G, Rong YS, McGinnis NM, Golic KG, Rio DC and Rubin GM (2004) _Drosophila melanogaster_ MNK/Chk2 and p53 regulate
multiple DNA repair and apoptotic pathways following DNA damage. _Mol. Cell. Biol._ 24: 1219–1231. CAS PubMed PubMed Central Google Scholar * Peters M, DeLuca C, Hirao A, Stambolic V,
Potter J, Zhou L, Liepa J, Snow B, Arya S, Wong J, Bouchard D, Binari R, Manoukian AS and Mak TW (2002) Chk2 regulates irradiation-induced, p53-mediated apoptosis in _Drosophila_. _Proc.
Natl. Acad. Sci. USA_ 99: 11305–11310. CAS PubMed PubMed Central Google Scholar * Harms KL and Chen X (2005) The C terminus of p53 family proteins is a cell fate determinant. _Mol. Cell.
Biol._ 25: 2014–2030. CAS PubMed PubMed Central Google Scholar * Chao C, Saito S, Kang J, Anderson CW, Appella E and Xu Y (2000) p53 transcriptional activity is essential for
p53-dependent apoptosis following DNA damage. _EMBO J._ 19: 4967–4975. CAS PubMed PubMed Central Google Scholar * Jimenez GS, Nister M, Stommel JM, Beeche M, Barcarse EA, Zhang XQ,
O'Gorman S and Wahl GM (2000) A transactivation-deficient mouse model provides insights into Trp53 regulation and function. _Nat. Genet._ 26: 37–43. CAS PubMed Google Scholar *
Johnson TM, Hammond EM, Giaccia A and Attardi LD (2005) The p53QS transactivation-deficient mutant shows stress-specific apoptotic activity and induces embryonic lethality. _Nat. Genet._ 37:
145–152. CAS PubMed Google Scholar * Nister M, Tang M, Zhang XQ, Yin C, Beeche M, Hu X, Enblad G, van Dyke T and Wahl GM (2005) p53 must be competent for transcriptional regulation to
suppress tumor formation. _Oncogene_ 24: 3563–3573. CAS PubMed Google Scholar * Tang M, Wahl GM and Nister M (2006) Explaining the biological activity of transactivation-deficient p53
variants. _Nat. Genet._ 38: 395–396. PubMed Google Scholar * Espinosa JM and Emerson BM (2001) Transcriptional regulation by p53 through intrinsic DNA/chromatin binding and site-directed
cofactor recruitment. _Mol. Cell_ 8: 57–69. CAS PubMed Google Scholar * Nakamura S, Roth JA and Mukhopadhyay T (2000) Multiple lysine mutations in the C-terminal domain of p53 interfere
with MDM2-dependent protein degradation and ubiquitination. _Mol. Cell. Biol._ 20: 9391–9398. CAS PubMed PubMed Central Google Scholar * Rodriguez MS, Desterro JM, Lain S, Lane DP and
Hay RT (2000) Multiple C-terminal lysine residues target p53 for ubiquitin-proteasome-mediated degradation. _Mol. Cell. Biol._ 20: 8458–8467. CAS PubMed PubMed Central Google Scholar *
Krummel KA, Toledo F, Lee CJ and Wahl GM (2005) The C-terminal lysines fine-tune p53 stress responses in a mouse model, but are not required for stability control or transactivation. _Proc.
Natl. Acad. Sci. USA_ 102: 10188–10193. CAS PubMed PubMed Central Google Scholar * Feng L, Lin T, Uranishi H, Gu W and Xu Y (2005) Functional analysis of the roles of posttranslational
modifications at the p53 C terminus in regulating p53 stability and activity. _Mol. Cell. Biol._ 25: 5389–5395. CAS PubMed PubMed Central Google Scholar * Gu J, Nie L, Wiederschain D and
Yuan ZM (2001) Identification of p53 sequence elements that are required for MDM2-mediated nuclear export. _Mol. Cell. Biol._ 21: 8533–8546. CAS PubMed PubMed Central Google Scholar *
Grossman SR, Deato ME, Brignone C, Chan HM, Kung AL, Tagami H, Nakatani Y and Livingston DM (2003) Polyubiquitination of p53 by a ubiquitin ligase activity of p300. _Science_ 300: 342–344.
CAS PubMed Google Scholar * Balint E, Bates S and Vousden KH (1999) Mdm2 binds p73 alpha without targeting degradation. _Oncogene_ 18: 3923–3929. CAS PubMed Google Scholar * Zeng X,
Chen L, Jost CA, Maya R, Keller D, Wang X, Kaelin Jr WG, Oren M, Chen J and Lu H (1999) MDM2 suppresses p73 function without promoting p73 degradation. _Mol. Cell. Biol._ 19: 3257–3266. CAS
PubMed PubMed Central Google Scholar * Brignone C, Bradley KE, Kisselev AF and Grossman SR (2004) A post-ubiquitination role for MDM2 and hHR23A in the p53 degradation pathway.
_Oncogene_ 23: 4121–4129. CAS PubMed Google Scholar * Glockzin S, Ogi FX, Hengstermann A, Scheffner M and Blattner C (2003) Involvement of the DNA repair protein hHR23 in p53 degradation.
_Mol. Cell. Biol._ 23: 8960–8969. CAS PubMed PubMed Central Google Scholar * Mendrysa SM, McElwee MK, Michalowski J, O'Leary KA, Young KM and Perry ME (2003) Mdm2 is critical for
inhibition of p53 during lymphopoiesis and the response to ionizing irradiation. _Mol. Cell. Biol._ 23: 462–472. CAS PubMed PubMed Central Google Scholar * Alt JR, Greiner TC, Cleveland
JL and Eischen CM (2003) Mdm2 haplo-insufficiency profoundly inhibits Myc-induced lymphomagenesis. _EMBO J._ 22: 1442–1450. CAS PubMed PubMed Central Google Scholar * Bond GL, Hu W, Bond
EE, Robins H, Lutzker SG, Arva NC, Bargonetti J, Bartel F, Taubert H, Wuerl P, Onel K, Yip L, Hwang SJ, Strong LC, Lozano G and Levine AJ (2004) A single nucleotide polymorphism in the MDM2
promoter attenuates the p53 tumor suppressor pathway and accelerates tumor formation in humans. _Cell_ 119: 591–602. CAS PubMed Google Scholar * Dumaz N, Milne DM, Jardine LJ and Meek DW
(2001) Critical roles for the serine 20, but not the serine 15, phosphorylation site and for the polyproline domain in regulating p53 turnover. _Biochem. J._ 359 (Part 2): 459–464. CAS
PubMed PubMed Central Google Scholar * Liu G, Xia T and Chen X (2003) The activation domains, the proline-rich domain, and the C-terminal basic domain in p53 are necessary for acetylation
of histones on the proximal p21 promoter and interaction with p300/CBP. _J. Biol. Chem._ 278: 17557–17565. CAS PubMed Google Scholar * Chang NS, Pratt N, Heath J, Schultz L, Sleve D,
Carey GB and Zevotek N (2001) Hyaluronidase induction of a WW domain-containing oxidoreductase that enhances tumor necrosis factor cytotoxicity. _J. Biol. Chem._ 276: 3361–3370. CAS PubMed
Google Scholar * Zilfou JT, Hoffman WH, Sank M, George DL and Murphy M (2001) The corepressor mSin3a interacts with the proline-rich domain of p53 and protects p53 from
proteasome-mediated degradation. _Mol. Cell. Biol._ 21: 3974–3985. CAS PubMed PubMed Central Google Scholar * Walker KK and Levine AJ (1996) Identification of a novel p53 functional
domain that is necessary for efficient growth suppression. _Proc. Natl. Acad. Sci. USA_ 93: 15335–15340. CAS PubMed PubMed Central Google Scholar * Sionov RV and Haupt Y (1999) The
cellular response to p53: the decision between life and death. _Oncogene_ 18: 6145–6157. CAS PubMed Google Scholar * Venot C, Maratrat M, Dureuil C, Conseiller E, Bracco L and Debussche L
(1998) The requirement for the p53 proline-rich functional domain for mediation of apoptosis is correlated with specific PIG3 gene transactivation and with transcriptional repression. _EMBO
J._ 17: 4668–4679. CAS PubMed PubMed Central Google Scholar * Zhu J, Jiang J, Zhou W, Zhu K and Chen X (1999) Differential regulation of cellular target genes by p53 devoid of the PXXP
motifs with impaired apoptotic activity. _Oncogene_ 18: 2149–2155. CAS PubMed Google Scholar * Bell DW, Varley JM, Szydlo TE, Kang DH, Wahrer DC, Shannon KE, Lubratovich M, Verselis SJ,
Isselbacher KJ, Fraumeni JF, Birch JM, Li FP, Garber JE and Haber DA (1999) Heterozygous germ line hCHK2 mutations in Li–Fraumeni syndrome. _Science_ 286: 2528–2531. CAS PubMed Google
Scholar * Takai H, Naka K, Okada Y, Watanabe M, Harada N, Saito S, Anderson CW, Appella E, Nakanishi M, Suzuki H, Nagashima K, Sawa H, Ikeda K and Motoyama N (2002) Chk2-deficient mice
exhibit radioresistance and defective p53-mediated transcription. _EMBO J._ 21: 5195–5205. CAS PubMed PubMed Central Google Scholar * Sun XF, Johannsson O, Hakansson S, Sellberg G,
Nordenskjold B, Olsson H and Borg A (1996) A novel p53 germline alteration identified in a late onset breast cancer kindred. _Oncogene_ 13: 407–411. CAS PubMed Google Scholar * Marine JC
and Jochemsen AG (2004) Mdmx and Mdm2: brothers in arms? _Cell Cycle_ 3: 900–904. CAS PubMed Google Scholar * Marine JC and Jochemsen AG (2005) Mdmx as an essential regulator of p53
activity. _Biochem. Biophys. Res. Commun._ 331: 750–760. CAS PubMed Google Scholar * Jones SN, Roe AE, Donehower LA and Bradley A (1995) Rescue of embryonic lethality in Mdm2-deficient
mice by absence of p53. _Nature_ 378: 206–208. CAS PubMed Google Scholar * Montes de Oca Luna R, Wagner DS and Lozano G (1995) Rescue of early embryonic lethality in mdm2-deficient mice
by deletion of p53. _Nature_ 378: 203–206. CAS PubMed Google Scholar * Parant J, Chavez-Reyes A, Little NA, Yan W, Reinke V, Jochemsen AG and Lozano G (2001) Rescue of embryonic lethality
in Mdm4-null mice by loss of Trp53 suggests a nonoverlapping pathway with MDM2 to regulate p53. _Nat. Genet._ 29: 92–95. CAS PubMed Google Scholar * Finch RA, Donoviel DB, Potter D, Shi
M, Fan A, Freed DD, Wang CY, Zambrowicz BP, Ramirez-Solis R, Sands AT and Zhang N (2002) mdmx is a negative regulator of p53 activity _in vivo_. _Cancer Res._ 62: 3221–3225. CAS PubMed
Google Scholar * Migliorini D, Denchi EL, Danovi D, Jochemsen A, Capillo M, Gobbi A, Helin K, Pelicci PG and Marine JC (2002) Mdm4 (Mdmx) regulates p53-induced growth arrest and neuronal
cell death during early embryonic mouse development. _Mol. Cell. Biol._ 22: 5527–5538. CAS PubMed PubMed Central Google Scholar * Pomerantz J, Schreiber-Agus N, Liegeois NJ, Silverman A,
Alland L, Chin L, Potes J, Chen K, Orlow I, Lee HW, Cordon-Cardo C and DePinho RA (1998) The Ink4a tumor suppressor gene product, p19Arf, interacts with MDM2 and neutralizes MDM2's
inhibition of p53. _Cell_ 92: 713–723. CAS PubMed Google Scholar * Xirodimas D, Saville MK, Edling C, Lane DP and Lain S (2001) Different effects of p14ARF on the levels of ubiquitinated
p53 and Mdm2 _in vivo_. _Oncogene_ 20: 4972–4983. CAS PubMed Google Scholar * Higashitsuji H, Higashitsuji H, Itoh K, Sakurai T, Nagao T, Sumitomo Y, Masuda T, Dawson S, Shimada Y, Mayer
RJ and Fujita J (2005) The oncoprotein gankyrin binds to MDM2/HDM2, enhancing ubiquitylation and degradation of p53. _Cancer Cell_ 8: 75–87. CAS PubMed Google Scholar * Higashitsuji H,
Liu Y, Mayer RJ and Fujita J (2005) The oncoprotein gankyrin negatively regulates both p53 and RB by enhancing proteasomal degradation. _Cell Cycle_ 4: 1335–1337. CAS PubMed Google Scholar
* Li M, Brooks CL, Kon N and Gu W (2004) A dynamic role of HAUSP in the p53–Mdm2 pathway. _Mol. Cell_ 13: 879–886. CAS PubMed Google Scholar * Li M, Chen D, Shiloh A, Luo J, Nikolaev
AY, Qin J and Gu W (2002) Deubiquitination of p53 by HAUSP is an important pathway for p53 stabilization. _Nature_ 416: 648–653. CAS PubMed Google Scholar * Meulmeester E, Maurice MM,
Boutell C, Teunisse AF, Ovaa H, Abraham TE, Dirks RW and Jochemsen AG (2005) Loss of HAUSP-mediated deubiquitination contributes to DNA damage-induced destabilization of Hdmx and Hdm2. _Mol.
Cell_ 18: 565–576. CAS PubMed Google Scholar * Haupt Y, Maya R, Kazaz A and Oren M (1997) Mdm2 promotes the rapid degradation of p53. _Nature_ 387: 296–299. CAS PubMed Google Scholar
* Kubbutat MH, Jones SN and Vousden KH (1997) Regulation of p53 stability by Mdm2. _Nature_ 387: 299–303. CAS PubMed Google Scholar * Momand J, Zambetti GP, Olson DC, George D and Levine
AJ (1992) The mdm-2 oncogene product forms a complex with the p53 protein and inhibits p53-mediated transactivation. _Cell_ 69: 1237–1245. CAS PubMed Google Scholar * Lahav G, Rosenfeld
N, Sigal A, Geva-Zatorsky N, Levine AJ, Elowitz MB and Alon U (2004) Dynamics of the p53–Mdm2 feedback loop in individual cells. _Nat. Genet_ 36: 147–150. CAS PubMed Google Scholar * Ries
S, Biederer C, Woods D, Shifman O, Shirasawa S, Sasazuki T, McMahon M, Oren M and McCormick F (2000) Opposing effects of Ras on p53: transcriptional activation of mdm2 and induction of
p19ARF. _Cell_ 103: 321–330. CAS PubMed Google Scholar * Ashcroft M, Ludwig RL, Woods DB, Copeland TD, Weber HO, MacRae EJ and Vousden KH (2002) Phosphorylation of HDM2 by Akt. _Oncogene_
21: 1955–1962. CAS PubMed Google Scholar * Gottlieb TM, Leal JF, Seger R, Taya Y and Oren M (2002) Cross-talk between Akt, p53 and Mdm2: possible implications for the regulation of
apoptosis. _Oncogene_ 21: 1299–1303. CAS PubMed Google Scholar * Wood SA (2002) Dubble or nothing? Is HAUSP deubiquitylating enzyme the final arbiter of p53 levels? _Sci. STKE_ 2002
(143): pe34. PubMed Google Scholar * Cummings JM, Rago C, Kohli M, Kinzler KW, Lengauer C and Vogelstein B (2004) Tumour suppression: disruption of HAUSP gene stabilizes p53. _Nature_ 428:
486–487. Google Scholar * de Graaf P, Little NA, Ramos YF, Meulmeester E, Letteboer SJ and Jochemsen AG (2003) Hdmx protein stability is regulated by the ubiquitin ligase activity of Mdm2.
_J. Biol. Chem._ 278: 38315–38324. CAS PubMed Google Scholar * Kawai H, Wiederschain D, Kitao H, Stuart J, Tsai KK and Yuan ZM (2003) DNA damage-induced MDMX degradation is mediated by
MDM2. _J. Biol. Chem._ 278: 45946–45953. CAS PubMed Google Scholar * Meek DW and Knippschild U (2003) Posttranslational modification of MDM2. _Mol. Cancer Res._ 1: 1017–1026. CAS PubMed
Google Scholar * Pereg Y, Shkedy D, de Graaf P, Meulmeester E, Edelson-Averbukh M, Salek M, Biton S, Teunisse AF, Lehmann WD, Jochemsen AG and Shiloh Y (2005) Phosphorylation of Hdmx
mediates its Hdm2- and ATM-dependent degradation in response to DNA damage. _Proc. Natl. Acad. Sci. USA_ 102: 5056–5061. CAS PubMed PubMed Central Google Scholar * Stommel JM and Wahl GM
(2004) Accelerated MDM2 auto-degradation induced by DNA-damage kinases is required for p53 activation. _EMBO J._ 23: 1547–1556. CAS PubMed PubMed Central Google Scholar * Pan Y and Chen
J (2003) MDM2 promotes ubiquitination and degradation of MDMX. _Mol. Cell. Biol._ 23: 5113–5121. CAS PubMed PubMed Central Google Scholar * Kawai H, Wiederschain D and Yuan ZM (2003)
Critical contribution of the MDM2 acidic domain to p53 ubiquitination. _Mol. Cell. Biol._ 23: 4939–4947. CAS PubMed PubMed Central Google Scholar * Meulmeester E, Frenk R, Stad R, de
Graaf P, Marine JC, Vousden KH and Jochemsen AG (2003) Critical role for a central part of Mdm2 in the ubiquitylation of p53. _Mol. Cell. Biol._ 23: 4929–4938. CAS PubMed PubMed Central
Google Scholar * Tanimura S, Ohtsuka S, Mitsui K, Shirouzu K, Yoshimura A and Ohtsubo M (1999) MDM2 interacts with MDMX through their RING finger domains. _FEBS Lett._ 447: 5–9. CAS PubMed
Google Scholar * Chen L, Gilkes DM, Pan Y, Lane WS and Chen J (2005) ATM and Chk2-dependent phosphorylation of MDMX contribute to p53 activation after DNA damage. _EMBO J._ 24: 3411–3422.
CAS PubMed PubMed Central Google Scholar * Thompson T, Tovar C, Yang H, Carvajal D, Vu BT, Xu Q, Wahl GM, Heimbrook DC and Vassilev LT (2004) Phosphorylation of p53 on key serines is
dispensable for transcriptional activation and apoptosis. _J. Biol. Chem._ 279: 53015–53022. CAS PubMed Google Scholar Download references ACKNOWLEDGEMENTS I wish to thank all the members
of my lab, past and present, for their hard work and many animated discussions that made it possible to develop the models shown. Although Jayne Stommel is no longer in the lab, her
demonstration of damage-mediated destabilization of Mdm2 as an important early event in P53 activation and stabilization caused me to look at the problem very differently. The work of
Crystal Lee and Franck Toledo, who have also left recently, pointed out the importance of MdmX as a critical negative regulator of P53 transactivation in an _in vivo_ setting. Finally,
current members Kurt Krummel, Mark Wade, Vivian Wang, Ee-Tsin Wong, Leo Li, Stephanie Kinkel, and Mengjia Tang either provided data or suggestions that improved or clarified the manuscript.
This work has been supported by grants from the NCI (CA61449, CA48405) to GMW, MT, KK, and MW. AUTHOR INFORMATION AUTHORS AND AFFILIATIONS * Salk Institute for Biological Studies, Gene
Expression Laboratory, 10010 N. Torrey Pines Road, La Jolla, CA, USA G M Wahl Authors * G M Wahl View author publications You can also search for this author inPubMed Google Scholar
CORRESPONDING AUTHOR Correspondence to G M Wahl. ADDITIONAL INFORMATION Edited by G Melino RIGHTS AND PERMISSIONS Reprints and permissions ABOUT THIS ARTICLE CITE THIS ARTICLE Wahl, G. Mouse
bites dogma: how mouse models are changing our views of how P53 is regulated _in vivo_. _Cell Death Differ_ 13, 973–983 (2006). https://doi.org/10.1038/sj.cdd.4401911 Download citation *
Received: 04 January 2006 * Accepted: 20 February 2006 * Published: 31 March 2006 * Issue Date: 01 June 2006 * DOI: https://doi.org/10.1038/sj.cdd.4401911 SHARE THIS ARTICLE Anyone you share
the following link with will be able to read this content: Get shareable link Sorry, a shareable link is not currently available for this article. Copy to clipboard Provided by the Springer
Nature SharedIt content-sharing initiative KEYWORDS * p53 activation * mouse models * TP53