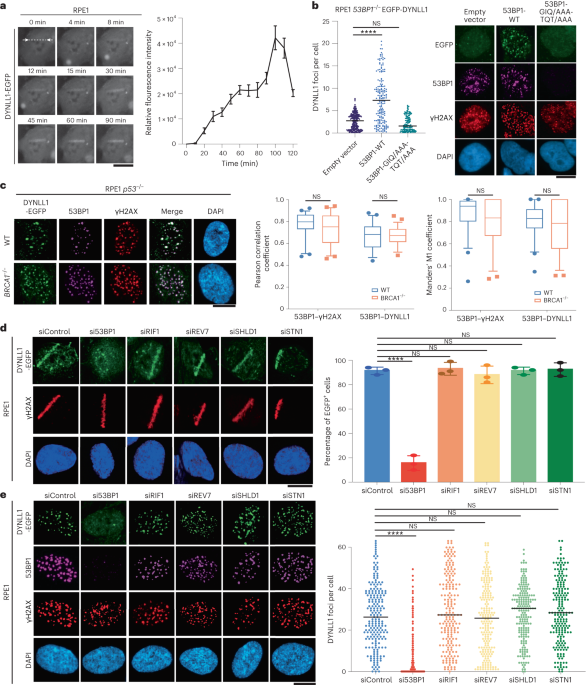
- Select a language for the TTS:
- UK English Female
- UK English Male
- US English Female
- US English Male
- Australian Female
- Australian Male
- Language selected: (auto detect) - EN
Play all audios:
ABSTRACT The extent and efficacy of DNA end resection at DNA double-strand breaks (DSB) determine the repair pathway choice. Here we describe how the 53BP1-associated protein DYNLL1 works in
tandem with the Shieldin complex to protect DNA ends. DYNLL1 is recruited to DSBs by 53BP1, where it limits end resection by binding and disrupting the MRE11 dimer. The Shieldin complex is
recruited to a fraction of 53BP1-positive DSBs hours after DYNLL1, predominantly in G1 cells. Shieldin localization to DSBs depends on MRE11 activity and is regulated by the interaction of
DYNLL1 with MRE11. BRCA1-deficient cells rendered resistant to PARP inhibitors by the loss of Shieldin proteins can be resensitized by the constitutive association of DYNLL1 with MRE11.
These results define the temporal and functional dynamics of the 53BP1-centric DNA end resection factors in cells. Access through your institution Buy or subscribe This is a preview of
subscription content, access via your institution ACCESS OPTIONS Access through your institution Access Nature and 54 other Nature Portfolio journals Get Nature+, our best-value
online-access subscription $29.99 / 30 days cancel any time Learn more Subscribe to this journal Receive 12 print issues and online access $209.00 per year only $17.42 per issue Learn more
Buy this article * Purchase on SpringerLink * Instant access to full article PDF Buy now Prices may be subject to local taxes which are calculated during checkout ADDITIONAL ACCESS OPTIONS:
* Log in * Learn about institutional subscriptions * Read our FAQs * Contact customer support SIMILAR CONTENT BEING VIEWED BY OTHERS PROMOTION OF DNA END RESECTION BY BRCA1–BARD1 IN
HOMOLOGOUS RECOMBINATION Article 11 September 2024 SHIELDIN COMPLEX ASSEMBLY KINETICS AND DNA BINDING BY SHLD3 Article Open access 08 April 2023 RIF1-ASF1-MEDIATED HIGH-ORDER CHROMATIN
STRUCTURE SAFEGUARDS GENOME INTEGRITY Article Open access 17 February 2022 DATA AVAILABILITY Numerical source data are provided with this paper. A description of the AlphaFold modeling can
be found in the Methods. All other data reported in this paper will be shared by the lead contact upon request. REFERENCES * Zhao, W., Wiese, C., Kwon, Y., Hromas, R. & Sung, P. The BRCA
tumor suppressor network in chromosome damage repair by homologous recombination. _Annu. Rev. Biochem._ 88, 221–245 (2019). Article CAS PubMed PubMed Central Google Scholar * Zhao, B.,
Rothenberg, E., Ramsden, D. A. & Lieber, M. R. The molecular basis and disease relevance of non-homologous DNA end joining. _Nat. Rev. Mol. Cell Biol._ 21, 765–781 (2020). Article CAS
PubMed PubMed Central Google Scholar * Hustedt, N. & Durocher, D. The control of DNA repair by the cell cycle. _Nat. Cell Biol._ 19, 1–9 (2016). Article PubMed Google Scholar *
Gnugge, R. & Symington, L. S. DNA end resection during homologous recombination. _Curr. Opin. Genet Dev._ 71, 99–105 (2021). Article CAS PubMed PubMed Central Google Scholar *
Bunting, S. F. et al. 53BP1 inhibits homologous recombination in Brca1-deficient cells by blocking resection of DNA breaks. _Cell_ 141, 243–254 (2010). Article CAS PubMed PubMed Central
Google Scholar * Bouwman, P. et al. 53BP1 loss rescues BRCA1 deficiency and is associated with triple-negative and BRCA-mutated breast cancers. _Nat. Struct. Mol. Biol._ 17, 688–695 (2010).
Article CAS PubMed PubMed Central Google Scholar * Mirman, Z. et al. 53BP1–RIF1–shieldin counteracts DSB resection through CST- and Polα-dependent fill-in. _Nature_ 560, 112–116
(2018). Article CAS PubMed PubMed Central Google Scholar * Zimmermann, M. & de Lange, T. 53BP1: pro choice in DNA repair. _Trends Cell Biol._ 24, 108–117 (2014). Article CAS
PubMed Google Scholar * Ochs, F. et al. 53BP1 fosters fidelity of homology-directed DNA repair. _Nat. Struct. Mol. Biol._ 23, 714–721 (2016). Article CAS PubMed Google Scholar *
Mirman, Z., Sasi, N. K., King, A., Chapman, J. R. & de Lange, T. 53BP1-shieldin-dependent DSB processing in BRCA1-deficient cells requires CST-Polalpha-primase fill-in synthesis. _Nat.
Cell Biol._ 24, 51–61 (2022). Article CAS PubMed PubMed Central Google Scholar * Mirman, Z. & de Lange, T. 53BP1: a DSB escort. _Genes Dev._ 34, 7–23 (2020). Article CAS PubMed
PubMed Central Google Scholar * Noordermeer, S. M. et al. The shieldin complex mediates 53BP1-dependent DNA repair. _Nature_ 560, 117–121 (2018). Article CAS PubMed PubMed Central
Google Scholar * Dev, H. et al. Shieldin complex promotes DNA end-joining and counters homologous recombination in BRCA1-null cells. _Nat. Cell Biol._ 20, 954–965 (2018). Article CAS
PubMed PubMed Central Google Scholar * Gupta, R. et al. DNA repair network analysis reveals shieldin as a key regulator of NHEJ and PARP inhibitor sensitivity. _Cell_ 173, 972–988.e23
(2018). Article CAS PubMed PubMed Central Google Scholar * He, Y. J. et al. DYNLL1 binds to MRE11 to limit DNA end resection in BRCA1-deficient cells. _Nature_ 563, 522–526 (2018).
Article CAS PubMed PubMed Central Google Scholar * Lo, K. W. et al. The 8-kDa dynein light chain binds to p53-binding protein 1 and mediates DNA damage-induced p53 nuclear accumulation.
_J. Biol. Chem._ 280, 8172–8179 (2005). Article CAS PubMed Google Scholar * West, K. L. et al. LC8/DYNLL1 is a 53BP1 effector and regulates checkpoint activation. _Nucleic Acids Res._
47, 6236–6249 (2019). Article CAS PubMed PubMed Central Google Scholar * Becker, J. R. et al. The ASCIZ-DYNLL1 axis promotes 53BP1-dependent non-homologous end joining and PARP
inhibitor sensitivity. _Nat. Commun._ 9, 5406 (2018). Article CAS PubMed PubMed Central Google Scholar * Setiaputra, D. et al. RIF1 acts in DNA repair through phosphopeptide recognition
of 53BP1. _Mol. Cell_ 82, 1359–1371 e9 (2022). Article CAS PubMed PubMed Central Google Scholar * Chen, H., Lisby, M. & Symington, L. S. RPA coordinates DNA end resection and
prevents formation of DNA hairpins. _Mol. Cell_ 50, 589–600 (2013). Article CAS PubMed Google Scholar * Cejka, P. DNA end resection: nucleases team up with the right partners to initiate
homologous recombination. _J. Biol. Chem._ 290, 22931–22938 (2015). Article CAS PubMed PubMed Central Google Scholar * Iacovoni, J. S. et al. High-resolution profiling of gammaH2AX
around DNA double strand breaks in the mammalian genome. _EMBO J._ 29, 1446–1457 (2010). Article CAS PubMed PubMed Central Google Scholar * Zhou, Y., Caron, P., Legube, G. & Paull,
T. T. Quantitation of DNA double-strand break resection intermediates in human cells. _Nucleic Acids Res._ 42, e19 (2014). Article CAS PubMed Google Scholar * Drane, P. et al. TIRR
regulates 53BP1 by masking its histone methyl-lysine binding function. _Nature_ 543, 211–216 (2017). Article CAS PubMed PubMed Central Google Scholar * Syed, A. & Tainer, J. A. The
MRE11-RAD50-NBS1 complex conducts the orchestration of damage signaling and outcomes to stress in DNA replication and repair. _Annu. Rev. Biochem._ 87, 263–294 (2018). Article CAS PubMed
PubMed Central Google Scholar * Hopfner, K. P. et al. Structural biochemistry and interaction architecture of the DNA double-strand break repair Mre11 nuclease and Rad50-ATPase. _Cell_
105, 473–485 (2001). Article CAS PubMed Google Scholar * Lammens, K. et al. The Mre11:Rad50 structure shows an ATP-dependent molecular clamp in DNA double-strand break repair. _Cell_
145, 54–66 (2011). Article CAS PubMed PubMed Central Google Scholar * Schiller, C. B. et al. Structure of Mre11-Nbs1 complex yields insights into ataxia-telangiectasia-like disease
mutations and DNA damage signaling. _Nat. Struct. Mol. Biol._ 19, 693–700 (2012). Article CAS PubMed PubMed Central Google Scholar * Park, Y. B., Chae, J., Kim, Y. C. & Cho, Y.
Crystal structure of human Mre11: understanding tumorigenic mutations. _Structure_ 19, 1591–1602 (2011). Article CAS PubMed Google Scholar * Jerabek-Willemsen, M., Wienken, C. J., Braun,
D., Baaske, P. & Duhr, S. Molecular interaction studies using microscale thermophoresis. _Assay. Drug Dev. Technol._ 9, 342–353 (2011). Article CAS PubMed PubMed Central Google
Scholar * Paull, T. T. 20 Years of Mre11 biology: no end in sight. _Mol. Cell_ 71, 419–427 (2018). Article CAS PubMed Google Scholar * Paull, T. T. & Lee, J. H. The Mre11/Rad50/Nbs1
complex and its role as a DNA double-strand break sensor for ATM. _Cell Cycle_ 4, 737–740 (2005). Article CAS PubMed Google Scholar * de Lange, T. Shelterin-mediated telomere
protection. _Annu. Rev. Genet._ 52, 223–247 (2018). Article PubMed Google Scholar * Maciejowski, J. & de Lange, T. Telomeres in cancer: tumour suppression and genome instability.
_Nat. Rev. Mol. Cell Biol._ 18, 175–186 (2017). Article CAS PubMed PubMed Central Google Scholar * Sakaue-Sawano, A. et al. Visualizing spatiotemporal dynamics of multicellular
cell-cycle progression. _Cell_ 132, 487–498 (2008). Article CAS PubMed Google Scholar * Biehs, R. et al. DNA double-strand break resection occurs during non-homologous end joining in G1
but is distinct from resection during homologous recombination. _Mol. Cell_ 65, 671–684 e5 (2017). Article CAS PubMed PubMed Central Google Scholar * Ye, Z. et al. GRB2 enforces
homology-directed repair initiation by MRE11. _Sci. Adv._ 7, eabe9254 (2021). Article CAS PubMed PubMed Central Google Scholar * Stracker, T. H. & Petrini, J. H. The MRE11 complex:
starting from the ends. _Nat. Rev. Mol. Cell Biol._ 12, 90–103 (2011). Article CAS PubMed PubMed Central Google Scholar * Setiaputra, D. & Durocher, D. Shieldin - the protector of
DNA ends. _EMBO Rep._ 20, e47560 (2019). Article PubMed PubMed Central Google Scholar * Zhao, F. et al. ASTE1 promotes shieldin complex mediated DNA repair by attenuating end resection.
_Nat. Cell Biol._ 23, 894–904 (2021). Article CAS PubMed Google Scholar * Cantor, S. B. Revisiting the BRCA-pathway through the lens of replication gap suppression: ‘gaps determine
therapy response in BRCA mutant cancer’. _DNA Repair_ 107, 103209 (2021). Article CAS PubMed PubMed Central Google Scholar * Paniagua, I. et al. MAD2L2 promotes replication fork
protection and recovery in a shieldin-independent and REV3L-dependent manner. _Nat. Commun._ 13, 5167 (2022). Article CAS PubMed PubMed Central Google Scholar * Lyu, X. et al. Human CST
complex protects stalled replication forks by directly blocking MRE11 degradation of nascent-strand DNA. _EMBO J._ 40, e103654 (2021). Article CAS PubMed Google Scholar * Sanjana, N.
E., Shalem, O. & Zhang, F. Improved vectors and genome-wide libraries for CRISPR screening. _Nat. Methods_ 11, 783–784 (2014). Article CAS PubMed PubMed Central Google Scholar *
Rosenberg, D. J., Syed, A., Tainer, J. A. & Hura, G. L. Monitoring nuclease activity by X-Ray scattering interferometry using gold nanoparticle-conjugated DNA. _Methods Mol. Biol._ 2444,
183–205 (2022). Article PubMed PubMed Central Google Scholar * Schneidman-Duhovny, D., Hammel, M., Tainer, J. A. & Sali, A. FoXS, FoXSDock and MultiFoXS: single-state and
multi-state structural modeling of proteins and their complexes based on SAXS profiles. _Nucleic Acids Res._ 44, W424–W429 (2016). Article CAS PubMed PubMed Central Google Scholar *
Rosenberg, D. J., Hura, G. L. & Hammel, M. Size exclusion chromatography coupled small angle X-ray scattering with tandem multiangle light scattering at the SIBYLS beamline. _Methods
Enzymol._ 677, 191–219 (2022). Article CAS PubMed Google Scholar * Hura, G. L. et al. Robust, high-throughput solution structural analyses by small angle X-ray scattering (SAXS). _Nat.
Methods_ 6, 606–612 (2009). Article CAS PubMed PubMed Central Google Scholar * Mirdita, M. et al. ColabFold: making protein folding accessible to all. _Nat. Methods_ 19, 679–682 (2022).
Article CAS PubMed PubMed Central Google Scholar * Schneidman-Duhovny, D., Hammel, M., Tainer, J. A. & Sali, A. Accurate SAXS profile computation and its assessment by contrast
variation experiments. _Biophys. J._ 105, 962–974 (2013). Article CAS PubMed PubMed Central Google Scholar Download references ACKNOWLEDGEMENTS D.C. is supported by grants R01 CA208244
and R01 CA264900, Gray Foundation Team Science Award, DOD Ovarian Cancer Award W81XWH-15-0564/OC140632, Tina’s Wish Foundation, Detect Me If You Can, V Foundation Award and the Claudia Adams
Barr Program in Innovative Basic Cancer Research. M.L.S. is supported by National Institutes of Health (NIH) grant F32 GM149115. J.A.T. and A.S. were partly supported by NIH grants P01
CA092548 and R35 CA220430, plus Cancer Prevention Research Institute of Texas grant RP180813 and an endowed Robert A. Welch Chemistry Chair supported by the Welch Foundation. B.T. was
supported by the Polish National Agency for Academic Exchange (grant PPN/WAL/2019/1/00018) and by the Foundation for Polish Science (START Program). We thank M. Hammel and K. H. Burnett for
their help in SEC–SAXS data collection at the SIBYLS beamline. The SEC–SAXS data collection at SIBYLS is supported in part by the NIH-NIGMS grant P30 GM124169-01 (ALS-ENABLE). We thank the
Center for Macromolecular Interactions at Harvard Medical School for access to their Monolith NT.115Pico (NanoTemper) system. We also thank J. Newman for providing the MRE11 expression
construct to produce the recombinant protein. The model in Fig. 8 was created with BioRender.com. AUTHOR INFORMATION Author notes * These authors contributed equally: Michelle L. Swift, Rui
Zhou. AUTHORS AND AFFILIATIONS * Division of Radiation and Genome Stability, Department of Radiation Oncology, Dana-Farber Cancer Institute, Harvard Medical School, Boston, MA, USA Michelle
L. Swift, Rui Zhou, Aleem Syed, Lisa A. Moreau, Alan D. D’Andrea, Yizhou Joseph He & Dipanjan Chowdhury * Cancer Center, Union Hospital, Tongji Medical College, Huazhong University of
Science and Technology, Wuhan, China Rui Zhou * Institute of Radiation Oncology, Union Hospital, Tongji Medical College, Huazhong University of Science and Technology, Wuhan, China Rui Zhou
* Center for DNA Damage and Repair, Dana-Farber Cancer Institute, Harvard Medical School, Boston, MA, USA Lisa A. Moreau & Alan D. D’Andrea * Department of Biostatistics and
Translational Medicine, Medical University of Łódź, Łódź, Poland Bartłomiej Tomasik * Department of Oncology and Radiotherapy, Medical University of Gdańsk, Faculty of Medicine, Gdańsk,
Poland Bartłomiej Tomasik * Molecular Biophysics and Integrated Bioimaging, Lawrence Berkeley National Laboratory, Berkeley, CA, USA John A. Tainer * Department of Molecular and Cellular
Oncology and Department of Cancer Biology, The University of Texas MD Anderson Cancer Center, Houston, TX, USA John A. Tainer * Department of Medical Oncology, Dana-Farber Cancer Institute,
Harvard Medical School, Boston, MA, USA Panagiotis A. Konstantinopoulos * Broad Institute of Harvard and MIT, Cambridge, MA, USA Dipanjan Chowdhury * Department of Biological Chemistry &
Molecular Pharmacology, Harvard Medical School, Boston, MA, USA Dipanjan Chowdhury Authors * Michelle L. Swift View author publications You can also search for this author inPubMed Google
Scholar * Rui Zhou View author publications You can also search for this author inPubMed Google Scholar * Aleem Syed View author publications You can also search for this author inPubMed
Google Scholar * Lisa A. Moreau View author publications You can also search for this author inPubMed Google Scholar * Bartłomiej Tomasik View author publications You can also search for
this author inPubMed Google Scholar * John A. Tainer View author publications You can also search for this author inPubMed Google Scholar * Panagiotis A. Konstantinopoulos View author
publications You can also search for this author inPubMed Google Scholar * Alan D. D’Andrea View author publications You can also search for this author inPubMed Google Scholar * Yizhou
Joseph He View author publications You can also search for this author inPubMed Google Scholar * Dipanjan Chowdhury View author publications You can also search for this author inPubMed
Google Scholar CONTRIBUTIONS M.L.S., R.Z. and Y.J.H. performed most of the experiments. A.S. purified recombinant proteins, designed and performed MST assays, and performed SEC–SAXS analyses
and structural modeling. L.A.M. performed metaphase spreads and radial formation assay. B.T. generated plasmids and created cell lines. Y.J.H., J.A.T., A.D.D., P.A.K. and D.C. conceived the
study. D.C. and M.L.S. wrote the manuscript with contributions from all authors. CORRESPONDING AUTHORS Correspondence to Yizhou Joseph He or Dipanjan Chowdhury. ETHICS DECLARATIONS
COMPETING INTERESTS A.D.D. reports consulting for AstraZeneca, Bayer AG, Blacksmith/Lightstone Ventures, Bristol Myers Squibb, Cyteir Therapeutics, EMD Serono, Impact Therapeutics, PrimeFour
Therapeutics, Pfizer, Tango Therapeutics and Zentalis Pharmaceuticals/Zeno Management; is an Advisory Board member for Cyteir and Impact Therapeutics; a stockholder in Cedilla Therapeutics,
Cyteir, Impact Therapeutics and PrimeFour Therapeutics, and reports receiving commercial research grants from Bristol Myers Squibb, EMD Serono, Moderna and Tango Therapeutics. The remaining
authors declare no competing interests. PEER REVIEW PEER REVIEW INFORMATION _Nature Structural & Molecular Biology_ thanks the anonymous reviewers for their contribution to the peer
review of this work. Peer reviewer reports are available. Dimitris Typas was the primary editor on this article and managed its editorial process and peer review in collaboration with the
rest of the editorial team. ADDITIONAL INFORMATION PUBLISHER’S NOTE Springer Nature remains neutral with regard to jurisdictional claims in published maps and institutional affiliations.
EXTENDED DATA EXTENDED DATA FIG. 1 53BP1 IS NECESSARY FOR CHROMATIN LOCALIZATION OF DYNLL1. RELATED TO FIG. 1. (A) Representative immunofluorescence images of RPE1 cells subjected to laser
microirradiation. Cells were fixed at indicated time points post laser microirradiation and processed for immunofluorescence with DYNLL1 and 53BP1 antibodies. (B) Representative images of
RPE1 wild-type or 53BP1−/− cells 2 h after exposure to 2 Gy irradiation or laser microirradiation. Cells were fixed and processed for immunofluorescence using antibodies against 53BP1, GFP
(DYNLL1), and γH2AX. (C) RPE1 cells depleted of p53, 53BP1, or DYNLL1 using CRISPR/Cas9 were exposed to 10 Gy irradiation. Protein was collected after 3 h. Localization of DYNLL1 to
chromatin was evaluated by subcellular fractionation followed by immunoblotting for DYNLL1. (D–F) Representative images of COV362 cells (D) and COV362 wild-type or 53BP1−/− cells (E, F)
exposed to 2 Gy irradiation (D, E) or laser microirradiation (F). 2 h post-recovery cells were fixed and processed for immunofluorescence using antibodies against 53BP1, GFP (DYNLL1), and
γH2AX. Box plots show mean and center, quartiles (boxes), and range (whiskers)(D). (G) Representative immunofluorescence images of RPE1 cells depleted of DYNLL1 using CRISPR/Cas9 and exposed
to 2 Gy irradiation. 2 h post-irradiation, cells were fixed and processed for immunofluorescence using antibodies against 53BP1 and γH2AX. (A-G) n = 3 biologically independent experiments,
counting ≥ 100 cells per experiment. Error bars represent the mean±s.e.m. P-values for foci quantification and "laser positive" cell analysis were calculated using two-sided
unpaired t-tests. P values are indicated by nonsignificant (P >0.05), *(P < 0.05), **(P < 0.01), ***(P < 0.001), ****(P<0.0001). Black line in dot plots represent median.
Scale bar = 20μm. Source data EXTENDED DATA FIG. 2 FORCE TETHERING DYNLL1 TO CHROMATIN INHIBITS MRE11 FOCI FORMATION. RELATED TO FIG. 2. (A, B) RPE1 53BP1−/− cells were transfected with
EGFP-tagged DYNLL1 or DYNLL1-FHA constructs. Cells were subjected to 2 Gy irradiation (A) or laser microirradiation (B). 2 h later cells were fixed and processed for immunofluorescence using
antibodies against GFP (DYNLL1) and γH2AX. (C, D) COV362 53BP1−/− cells were transfected with a EGFP-tagged DYNLL1 and DYNLL1-FHA constructs. Cells were subjected to 2 Gy irradiation (C) or
laser microirradiation (D). 2 h later cells were fixed and processed for immunofluorescence using antibodies against GFP (DYNLL1) and γH2AX. (E) COV362 53BP1−/− cells were transfected with
EGFP-tagged DYNLL1 and DYNLL1-FHA constructs. Cells were exposed to 2 Gy irradiation. 2 h post-irradiation cells were fixed and processed for immunofluorescence using antibodies against
MRE11, GFP (DYNLL1) and γH2AX. (A-E) n = 3 biologically independent experiments, counting ≥ 100 cells per experiment. Error bars represent the mean±s.e.m. P-values for foci quantification
and "laser positive" cell analysis were calculated using two-sided unpaired t-tests. P values are indicated by nonsignificant (P >0.05), *(P < 0.05), **(P < 0.01), ***(P
< 0.001), ****(P<0.0001). Black line in dot plots represent median. Scale bar = 20μm. Source data EXTENDED DATA FIG. 3 DYNLL1 CHROMATIN BINDING SUPPRESSES 53BP1 LOSS-INDUCED
RESTORATION OF HR IN BRCA1 DEFICIENT CELLS. RELATED TO FIG. 3. (A) MEFs expressing EGFP-tagged DYNLL1, and EGFP-tagged DYNLL1-FHA domains constructs were exposed to 2 Gy irradiation. 2 h
after irradiation cells were fixed and processed for immunofluorescence using a GFP (DYNLL1) antibody. (B, C) MEF p53−/− BRCA1−/− 53BP1−/− cells (B) and COV362 53BP1−/− cells (C) were
transfected with EGFP-tagged DYNLL, or EGFP-tagged DYNNL1-FHA constructs. Cells were exposed to 2 Gy irradiation. 2 h later, cells were fixed and processed for immunofluorescence using
antibodies against GFP (DYNLL1), RAD51, and γH2AX. (D) COV362 53BP1−/− cells were transfected EGFP-tagged DYNLL, or EGFP-tagged DYNNL1-FHA constructs. Cells were treated with indicated
concentrations of Olaparib for 6 days. Percent survival was determined via a cell viability assay. (A-D) n = 3 biologically independent experiments, counting ≥ 100 cells per experiment.
Error bars represent the mean±s.e.m. P-values for foci quantification were calculated using two-sided unpaired t-tests. P-value measurements for cell survival curves were assessed by
non-regression curve analysis. P values are indicated by nonsignificant (P >0.05), *(P < 0.05), **(P < 0.01), ***(P < 0.001), ****(P<0.0001). Black line in dot plots represent
median. Scale bar = 20μm. Source data EXTENDED DATA FIG. 4 DYNLL1 INTERFERES WITH MRE11 DIMERIZATION. RELATED TO FIG. 4. (A) Predicted structure of full-length MRE11 created by AlphaFold
Monomer V2 for Uniprot Accession number P49959. The structured catalytic domain of MRE11 is highlighted with a red circle. The model is color coded in terms of confidence in prediction and
respective color schemes for the confidence is given in the figure. In general, disordered regions have less confidence in model prediction, thus indicating the unstructured regions of MRE11
beyond capping domain. (B) Coomassie-stained protein gels indicating the quality of the recombinant protein used in the current study. Left: DYNLL1 mutants after cleaving the His-tag with
TEV protease. Right: MRE11 catalytic domain after the gel-filtration purification step. The red rectangle indicates the fractions that are combined. M indicates the protein standards and *
indicates the MRE11 degradation bands. (C) Average MST response (n = 3) measured from labeled MRE11 in the MST buffer or MST buffer with 5 μM DYNLL1-S88A or DYNLL1-S88D mutant. (D) Change in
the normalized fluorescence (ΔFnorm) as result of thermophoresis in the MST experiment plotted as a function of concentration of unlabeled MRE11. The resulting curves represents MRE11
dimerization in the absence of any DYNLL1 (black circles), in the presence of 500 nM DYNLL1-S88D (red triangles) or in the presence of 5 _μ_M DYNLL1-S88D (blue squares). The _K_d values are
measured by fitting the curves with _K_d model in the analysis software. (B-D) The data points represent average of three independent measurements and error bars represents standard
deviation. Source data EXTENDED DATA FIG. 5 SOLUTION STRUCTURES OF DYNLL1-WT AND MUTANTS. (A) AlphaFold2 predicted models of DYNLL1 dimer (left) and monomer (right). (B) Size exclusion
chromatography elution profiles for DYNLL1-WT, S88A and S88D mutants. For clarity, initial 8 mL (pre-void volume with no peaks) were omitted from the chromatograms. (C–E) FoXS fitting of
experimental X-ray scattering data (red dots) to theoretical SAXS profiles (solid lines) derived from structural models of DYNLL1 monomer (M) and dimer (D) or mixture of monomer and dimer
state. The goodness of the fit is evaluated by χ2. Guinier plots from the measured scattering intensity (I(Q)) as a function of scattering vector (Q) in the low Q region shown as insets for
WT and mutant proteins. Source data EXTENDED DATA FIG. 6 DEPLETION OF THE SHIELDIN COMPLEX DOES NOT AFFECT MRE11 RECRUITMENT. RELATED TO FIG. 5. (A) Protein expression from lysates collected
from RPE1 wild-type or DYNLL1−/− cells 2 h after 2 Gy irradiation. (B) COV362 cells overexpressing EGFP-DYNLL1 and transfected with siRNA targeting either 53BP1, or SHLD1 were exposed to 2
Gy irradiation. Cells were fixed 2 h post exposure and processed for immunofluorescence using antibodies against GFP (DYNLL1), γH2AX, and MRE11. (A,B) n = 3 biologically independent
experiments, counting ≥ 100 cells per experiment. P-values for foci quantification were calculated using two-sided unpaired t-tests. P values are indicated by nonsignificant (P >0.05),
*(P < 0.05), **(P < 0.01), ***(P < 0.001), ****(P<0.0001). Black line in dot plots represent median. Scale bar = 20μm. Source data EXTENDED DATA FIG. 7 SHIELDIN IS RECRUITED TO
DSBS LATER THAN DYNLL1 AND IN G1 PHASE ONLY. RELATED TO FIG. 6. (A) Representative images from Fig. 6a. (B) Quantification of GFP positive stripes for cells expressing DYNLL1-EGFP or
SHLD1-EGFP after exposure to laser microirradiation and fixed at indicated time points. (C) Quantification of number of DYNLL1 foci for RPE1 cells transduced with lentivirus comprised of the
Fucci system reporter assay. Cells were exposed to 10 Gy irradiation, fixed 6 h later, and processed using antibodies against Geminin, Cdt1, and DYNLL1. (D) Representative images for Fig.
6c and Extended Data Fig. 6c. (E) Representative western blots showing knockdown of indicated proteins for Fig. 6d. (F) RPE1 BRCA1−/− were subjected to 10 Gy IR and 4 h later were fixed and
processed for immunofluorescence using antibodies against SHLD1, Cyclin A, and γH2AX. (A-F) n = 3 biologically independent experiments, counting ≥ 100 cells per experiment. Error bars
represent the mean±s.e.m. P-values for foci quantification and ‘laser positive’ cell analysis was calculated using two-sided unpaired t-tests. P values are indicated by nonsignificant (P
>0.05), *(P < 0.05), **(P < 0.01), ***(P < 0.001), ****(P<0.0001). Black line in dot plots represent median. Scale bar = 20μm. Source data EXTENDED DATA FIG. 8 SHIELDIN
FUNCTIONS DOWNSTREAM OF DYNLL1, BUT IS NOT DEPENDENT ON DYNLL1 FOR ITS LOCALIZATION TO CHROMATIN. RELATED TO FIG. 7. (A) RPE1 p53−/− and RPE1 p53−/− 53BP1−/− cells were transfected with
EGFP-tagged DYNLL1 or EGFP-tagged DYNLL1-FHA. Cells were then transfected with mCherry-SHLD1 and subjected to laser microirradiation. 2 h after laser microirradiation cells were fixed and
processed for immunofluorescence using antibodies against GFP (DYNLL1), mCherry (SHLD1), and γH2AX. n = 3 biologically independent experiments. Scale bar = 20μm. SUPPLEMENTARY INFORMATION
SUPPLEMENTARY INFORMATION Supplementary Table 1 and Fig. 1. REPORTING SUMMARY PEER REVIEW FILE SOURCE DATA SOURCE DATA FIG. 1 Statistical source data for Fig. 1. SOURCE DATA FIG. 2
Statistical source data for Fig. 2. SOURCE DATA FIG. 2 Uncropped western blots for Fig. 2. SOURCE DATA FIG. 3 Statistical source data for Fig. 3. SOURCE DATA FIG. 4 Statistical source data
for Fig. 4. SOURCE DATA FIG. 4 Uncropped western blots for Fig. 4. SOURCE DATA FIG. 5 Statistical source data for Fig. 5. SOURCE DATA FIG. 6 Statistical source data for Fig. 6. SOURCE DATA
FIG. 7 Statistical source data for Fig. 7 SOURCE DATA EXTENDED DATA FIG. 1 Statistical source data for Extended Data Fig. 1. SOURCE DATA EXTENDED DATA FIG. 2 Uncropped western blots for
Extended Data Fig. 2. SOURCE DATA EXTENDED DATA FIG. 2 Statistical source data for Extended Data Fig. 2. SOURCE DATA EXTENDED DATA FIG. 3 Statistical source data for Extended Data Fig. 3.
SOURCE DATA EXTENDED DATA FIG. 4 Statistical source data for Extended Data Fig. 4. SOURCE DATA EXTENDED DATA FIG. 4 Uncropped gels for Extended Data Fig. 4. SOURCE DATA EXTENDED DATA FIG. 5
Statistical source data for Extended Data Fig. 5. SOURCE DATA EXTENDED DATA FIG. 6 Statistical source data for Extended Data Fig. 6. SOURCE DATA EXTENDED DATA FIG. 6 Uncropped western blots
for Extended Data Fig. 6. SOURCE DATA EXTENDED DATA FIG. 7 Statistical source data for Extended Data Fig. 7. SOURCE DATA EXTENDED DATA FIG. 7 Uncropped western blots for Extended Data Fig.
7. RIGHTS AND PERMISSIONS Springer Nature or its licensor (e.g. a society or other partner) holds exclusive rights to this article under a publishing agreement with the author(s) or other
rightsholder(s); author self-archiving of the accepted manuscript version of this article is solely governed by the terms of such publishing agreement and applicable law. Reprints and
permissions ABOUT THIS ARTICLE CITE THIS ARTICLE Swift, M.L., Zhou, R., Syed, A. _et al._ Dynamics of the DYNLL1–MRE11 complex regulate DNA end resection and recruitment of Shieldin to DSBs.
_Nat Struct Mol Biol_ 30, 1456–1467 (2023). https://doi.org/10.1038/s41594-023-01074-9 Download citation * Received: 31 January 2023 * Accepted: 21 July 2023 * Published: 11 September 2023
* Issue Date: October 2023 * DOI: https://doi.org/10.1038/s41594-023-01074-9 SHARE THIS ARTICLE Anyone you share the following link with will be able to read this content: Get shareable link
Sorry, a shareable link is not currently available for this article. Copy to clipboard Provided by the Springer Nature SharedIt content-sharing initiative