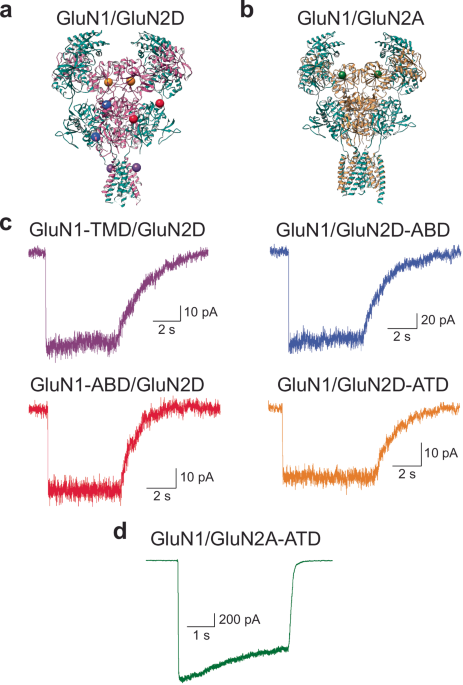
- Select a language for the TTS:
- UK English Female
- UK English Male
- US English Female
- US English Male
- Australian Female
- Australian Male
- Language selected: (auto detect) - EN
Play all audios:
ABSTRACT N-methyl-D-aspartate (NMDA) receptors are ionotropic glutamate receptors involved in learning and memory. NMDA receptors primarily comprise two GluN1 and two GluN2 subunits. The
GluN2 subunit dictates biophysical receptor properties, including the extent of receptor activation and desensitization. GluN2A- and GluN2D-containing receptors represent two functional
extremes. To uncover the conformational basis of their functional divergence, we utilize single-molecule fluorescence resonance energy transfer to probe the extracellular domains of these
receptor subtypes under resting and ligand-bound conditions. We find that the conformational profile of the GluN2 amino-terminal domain correlates with the disparate functions of GluN2A- and
GluN2D-containing receptors. Changes at the pre-transmembrane segments inversely correlate with those observed at the amino-terminal domain, confirming direct allosteric communication
between these domains. Additionally, binding of a positive allosteric modulator at the transmembrane domain shifts the conformational profile of the amino-terminal domain towards the active
state, revealing a bidirectional allosteric pathway between extracellular and transmembrane domains. SIMILAR CONTENT BEING VIEWED BY OTHERS CONFORMATIONAL REARRANGEMENT OF THE NMDA RECEPTOR
AMINO-TERMINAL DOMAIN DURING ACTIVATION AND ALLOSTERIC MODULATION Article Open access 11 May 2021 GLUN2A AND GLUN2B NMDA RECEPTORS USE DISTINCT ALLOSTERIC ROUTES Article Open access 05
August 2021 MOLECULAR MECHANISM OF LIGAND GATING AND OPENING OF NMDA RECEPTOR Article 31 July 2024 INTRODUCTION The majority of excitatory neurotransmission in the mammalian central nervous
system is accounted for by glutamatergic signaling. The ionotropic glutamate receptor family includes N-methyl-D-aspartate (NMDA), α-amino-3-hydroxy-5-methyl-4-isoxazolepropionic acid
(AMPA), kainate, and delta receptors1,2,3,4,5,6. NMDA receptor signaling is involved in learning, memory, and synaptic plasticity, while dysfunction of NMDA receptors has been implicated in
various neurological conditions, such as epilepsy, developmental delay, and ischemic stroke7,8,9. The NMDA receptor is a ligand-gated ion channel that requires simultaneous binding of
glutamate and co-agonist glycine to activate5,10,11. NMDA receptors adopt a heterotetrameric structure, with each receptor comprising two obligatory glycine-binding GluN1 subunits and two
glutamate-binding GluN2 (A-D) and/or glycine-binding GluN3 (A-B) subunits3,5,12,13,14,15. These various subunit types share a conserved architecture consisting of extracellular
amino-terminal (ATD) and agonist-binding domains (ABD), a transmembrane domain (TMD), and an intracellular carboxyl-terminal domain (CTD)5,12,13,16. The binding of glutamate and glycine to
the GluN2 and GluN1 agonist-binding domains induces conformational changes throughout the receptor, forming a transmembrane ion channel pore permeable to sodium, potassium, and calcium ions.
Several functional characteristics of NMDA receptors, including their extent of activation, desensitization profiles, and agonist potency, are determined by the identity of the GluN2
subunit17,18,19,20,21. GluN2A and GluN2D subunits represent opposite ends of the functional spectrum and differ in expression patterns. GluN1/GluN2A NMDA receptors are expressed broadly
throughout the cortex and hippocampus3,17,19. In contrast, GluN1/GluN2D NMDA receptors show broad expression in early development but later become restricted to distinct subgroups of
neurons, such as interneurons in the basal ganglia, hippocampus, and cerebellum3,17,19. Functionally, GluN1/GluN2A receptors demonstrate high open probabilities (~ 50%), fast deactivation (~
50 ms), and lower glutamate potency (EC50 of 3–5 µM). In comparison, GluN1/GluN2D receptors have low open probabilities (< 4%), slow deactivation kinetics (> 1 s), and high glutamate
potency (EC50 of 0.5 µM)20,22,23,24,25,26. A chimeric study found that replacing the GluN2A amino-terminal domain with that of GluN2D decreased open probabilities compared to GluN2A
wild-type receptors. In contrast, GluN2D receptor chimeras containing the GluN2A amino-terminal domain displayed increased open probabilities compared to wild-type GluN2D-containing
receptors22. These findings suggest that the amino-terminal domain contributes to the functional and kinetic differences observed between NMDA receptor subtypes22,27. While several cryo-EM
structures of the agonist-bound GluN1/GluN2A and GluN1/GluN2D receptors have been solved, the conformational differences underlying their differences in function could not be clearly
identified28,29,30,31,32. In addition, there are currently no apo state structures of the full-length GluN1/GluN2A or GluN1/GluN2D receptors. The lack of apo structures and unidentified
significant conformational differences between the two subtypes is likely due to the highly dynamic nature of GluN1/GluN2D receptors. Recent single-molecule FRET (smFRET) investigations have
determined the conformational landscape at the GluN1 amino-terminal domain33. However, recent structures of GluN2C-containing receptors reveal more significant conformational fluctuations
across the amino-terminal domains of GluN2 subunits28. Hence, studying the conformational landscape at the GluN2 subunits in conjunction with protein dynamics is essential to gaining a
comprehensive understanding of GluN2-dependent conformational variability underlying NMDA receptor subtype-specific gating properties. The functional properties of NMDA receptors have been
widely studied with respect to modulators31,34,35,36,37,38,39,40,41,42,43. Particularly, allosteric modulators have been observed to alter functional properties, such as open probability and
potentiation. While extensive insight has been gained into how modulators that bind at the amino-terminal domain control channel activity, much less is known about modulators binding to the
transmembrane segments31,34,35,36,37,38,39,40,41,42,43. The positive allosteric modulator GNE-9278 is particularly interesting as its binding site and functional properties have been
well-characterized39. GNE-9278 binds to the extracellular surface of the GluN1 pre-M1 transmembrane region and increases receptor currents in the presence of saturating concentrations of
glutamate and glycine. In addition, GNE-9278 slows deactivation kinetics and increases the potency of both glutamate and glycine. While GNE-9278 potentiates all GluN2-containing NMDA
receptors, the most significant effect is seen for GluN1/GluN2D receptors with an ~ 8–15-fold increase in currents (7.9 for HEK cells, 14.9 for oocytes)39. While the binding site of this
positive allosteric modulator has been determined, the mechanism underlying its function remains unclear. In this work, using single-molecule fluorescence resonance energy transfer (smFRET),
we investigate the full spectrum of conformational states occupied by GluN1/GluN2D receptors in apo and agonist-bound conditions in comparison to GluN1/GluN2A receptors. We employ smFRET to
measure distances across the GluN2 amino-terminal domain, the GluN1 and GluN2 agonist-binding domain clefts, and the GluN1 transmembrane domain. These measurements are used to categorize
and compare conformational states and state transitions at the millisecond time scale. Our results reveal that the GluN2D amino-terminal domain is inherently more decoupled and dynamic in
both apo and agonist-bound conditions. In contrast, the GluN1 transmembrane domain is more coupled and less dynamic in GluN1/GluN2D receptors than in GluN1/GluN2A receptors. The fraction of
GluN1/GluN2D receptors coupled at the amino-terminal domain is correlated with the fraction of the receptors exhibiting longer distances at the transmembrane segments. Furthermore, this
fraction also correlates with the low Popen of GluN1/GluN2D receptors, thereby providing a direct correlation between conformation and function of the receptor. In addition, we elucidate the
effects of GNE-9278 on the conformational and dynamic landscapes of the GluN1/GluN2D receptor to observe how the binding of an allosteric modulator to the pre-transmembrane region alters
allosteric communication between the transmembrane and amino-terminal domains. We find that GNE-9278 induces conformational changes far from its site of action by stabilizing the coupled
state of the GluN2D amino-terminal domain. This suggests that GNE-9278 demonstrates long-range bottom-up allostery in addition to a localized effect at the transmembrane segments. The
conformational landscape of the GluN1/GluN2D receptor in the presence of GNE-9278 resembles that observed for the GluN1/GluN2A receptor, suggesting a similarity in allosteric coupling
maintained across different GluN2 subunits and in the presence of small molecule modulators. RESULTS To study the conformational landscape of the extracellular domain of the GluN1/GluN2 NMDA
receptor, we utilized smFRET to examine distances throughout the receptor at both the GluN1 and GluN2 subunits. We designed four constructs that allowed us to measure inter-subunit
distances of the GluN2 amino-terminal domain and GluN1 transmembrane domain and distances at the GluN1 glycine-binding and GluN2 glutamate-binding domain clefts. We introduced donor-acceptor
fluorophores at specific sites within the protein using maleimide chemistry. To ensure specific labeling at our sites of interest, we mutated intrinsic non-disulfide bonded extracellular
cysteines (Cys15 and Cys22 in GluN1; Cys231, Cys399, and Cys460 in GluN2A; and Cys24, Cys245, and Cys485 in GluN2D) to serines to form cys-light GluN1 and GluN2 constructs. In addition, a
twin-strep tag was introduced at the C-terminus of GluN1 to facilitate the attachment of the heteromeric receptor to a microscope slide via streptavidin pull-down. For measuring changes near
the transmembrane domain (GluN1-TMD/GluN2D), we introduced a cysteine at site Phe554 in the GluN1 pre-M1 segment (Fig. 1a). To investigate changes across the GluN2D glutamate-binding domain
cleft (GluN1/GluN2D-ABD), we introduced cysteines at sites Gln528 and Met726 (Fig. 1a). For measuring changes across the GluN1 glycine-binding domain cleft (GluN1-ABD/GluN2D), we introduced
cysteines at sites Ser507 and Thr701 (Fig. 1a). For investigating changes across the GluN2 amino-terminal domain (GluN1/GluN2A-ATD and GluN1/GluN2D-ATD), we introduced a cysteine at site
Thr174 in GluN2A and Thr188 in GluN2D (Fig. 1a, b). Electrophysiological characterization of the above constructs demonstrated preserved activation, desensitization, and potentiation
function, validating their use in smFRET investigations (see Fig. 1c, d). To perform smFRET experiments, HEK293T cells were transfected with GluN1 constructs and either GluN2A or GluN2D
constructs (see “Methods” section for a detailed description). The NMDA receptors were labeled with donor (Alexa 555) and acceptor (Alexa 647) fluorophores, which have an R0 of 51 Å.
Subsequently, the labeled cells were solubilized and pulled down onto the smFRET slides. A confocal fluorescence microscope was used to visualize fluorophore-labeled receptors and select
receptors with one photobleaching FRET for analysis. Donor and acceptor fluorophore emissions were quantified by counting the number of photon detection events in 5 ms time bins, and the
FRET efficiency was calculated for each time bin. The resulting FRET traces from individual receptors across multiple independent biological replicates were combined and averaged into
cumulative FRET efficiency histograms with standard error bars representing the standard error across histograms from independent samples. Gaussian fitting guided by hidden Markov
model-based analysis of individual smFRET traces was used to determine the specific occupied FRET states and quantify the fraction of receptors occupying each FRET state44,45. RECEPTOR
ACTIVATION REFLECTED IN CONFORMATIONS NEAR PRE-M1 Functional studies have determined that GluN1/GluN2A and GluN1/GluN2D receptors vary in open probability, activation time course, and
desensitization profiles17,18,19,20,21. Thus, we began by examining conformational rearrangements across the transmembrane segments. We measured distances across the channel pore at the
GluN1 subunit pre-M1 segment in the unbound (apo) and glutamate and glycine-bound states. While structural studies have not been able to capture the apo conformation of NMDA receptors thus
far, we can assess the resting conformations of NMDA receptors with smFRET by applying glycine oxidase to remove contaminating glycine for the unliganded conditions, as previously performed
in Durham et al.46. Under apo conditions, we primarily observe a high FRET state of 0.97 (Fig. 2a and Table 1), which correlates to a FRET distance of ~ 29 Å. This distance agrees with the
published cryo-EM structure of the GluN1/GluN2D receptor bound by glycine and competitive antagonist CPP, where the distance between Phe554 on both GluN1 subunits is approximately 31 Å29.
This finding is consistent with the GluN1 transmembrane segments in the GluN1/GluN2D receptor being tightly packed and likely representing the closed, inactive channel state of the receptor.
The spread of states measured at the GluN1 pre-M1 segment demonstrates that the GluN2D-containing receptor is shifted to higher FRET states compared to the GluN1/GluN2A receptor, suggesting
an inherent difference at this site under resting conditions, with the GluN1/GluN2D receptor being more tightly packed (Fig. 2b)46,47. When both glutamate and glycine are bound to the
receptor (using saturating 1 mM concentrations of both ligands), we observe the FRET efficiencies are shifted lower than those observed under apo conditions (Fig. 2c, d). This likely
represents the pull on the transmembrane linker that contributes to the opening of the channel pore. However, we continue to observe a similar trend of GluN1/GluN2D receptors occupying a
higher FRET distribution compared to GluN1/GluN2A receptors, which is consistent with the lower open probabilities of this receptor subtype (Fig. 2d). The smFRET histograms were fit into
states based on Gaussian fitting guided by hidden Markov model-based analysis of the smFRET traces (Supplementary Fig. 1). Based on this analysis, we determined that in the unbound
condition, the GluN1/GluN2D receptor occupies primarily high FRET states of 0.78 and 0.97 (Table 1). Upon ligand binding, there is a ~ 6% occurrence of a low FRET efficiency of 0.59, which
indicates a proportion of receptors exploring longer distances across the transmembrane segments (Table 1). This 6% fraction is consistent with the Popen of less than 0.1 reported for
GluN1/GluN2D receptors, suggesting that this lower FRET efficiency state reflects channel opening. In addition, this fraction is significantly smaller than that observed for GluN1/GluN2A
receptors, where low FRET efficiencies compose ~ 35%, consistent with the higher Popen of approximately 0.5 for this subtype47. The smFRET traces also allow us to investigate conformational
dynamics in terms of transition probabilities between states. Transition probability per 5 ms was calculated based on the number of transitions observed in the raw smFRET data while taking
into consideration the relative occurrence of the starting state of each smFRET trace. The transition probability maps reveal that the transmembrane segments in both GluN1/GluN2A and
GluN1/GluN2D receptors are less dynamic in the apo conditions (Fig. 2e) than those observed in the fully liganded condition (Fig. 2f). This suggests that the transmembrane segments have
increased fluctuations when the receptor is bound by its ligands, allowing for the receptor to populate the low FRET efficiency activated state. In addition, we observe that the GluN1/GluN2A
transmembrane segments are significantly more dynamic than the GluN1/GluN2D transmembrane domain, suggesting a higher free energy barrier for the GluN1/GluN2D transmembrane segments to
transition into low FRET states46,47. This is consistent with the lower open probability of the GluN1/GluN2D receptor and further confirms that conformations arising from low FRET states
correlate with pore opening. AGONIST-INDUCED CHANGES AT THE LIGAND-BINDING DOMAINS To study the agonist-binding domain changes, we measured distances across the bilobed cleft in the GluN1
and GluN2 subunits. The smFRET histograms show that the unbound receptor occupies a broad spread of states at GluN1 and GluN2 agonist-binding clefts in the GluN1/GluN2D receptor (Figs.
3a–d). For both GluN2 and GluN1 agonist-binding clefts, two-sample _t_ tests comparing the means of the cumulative FRET efficiency histograms for GluN1/GluN2D and GluN1/GluN2A46 did not
return significant _p_-values. This suggests that the conformational landscape of the agonist-binding domain clefts is similar for both the GluN1/GluN2A and GluN1/GluN2D receptors in the apo
condition. Upon binding of glutamate and glycine, the distances across the cleft decrease, and the conformational spread is reduced at both GluN1 and GluN2D subunits (Fig. 3e–h). This is
consistent with agonist binding stabilizing the agonist-binding domains in a closed cleft conformation. Furthermore, the smFRET histograms for GluN1/GluN2D in the agonist-bound condition at
both clefts, as well as the states occupied (listed in Table 1, and Gaussian fits in Supplementary Fig. 1), are not significantly different from those previously observed for GluN1/GluN2A
receptors46,48,49. These studies reveal that the overall conformational landscape of the GluN1 and GluN2 agonist-binding domains are similar, both in the resting and agonist-bound forms
between GluN1/GluN2D and GluN1/GluN2A receptors. GLUN2-DEPENDENT CONFORMATIONS OF THE AMINO-TERMINAL DOMAIN Amino-terminal domain conformations dictate differences in receptor
activation22,50. Decoupling of the amino-terminal domain upon antagonist or inhibitor binding correlates with lower activity of GluN1/GluN2A and GluN1/GluN2B receptors29,51. To test if a
similar shift in conformational landscape underlies lower activity of the GluN1/GluN2D receptor, we studied distances across the GluN2 subunit amino-terminal domain in GluN2D- and
GluN2A-containing receptors. The smFRET data reveal a significant trend of lower FRET efficiencies in the apo (Fig. 4a, b) and agonist-bound conditions (Fig. 4c, d) for GluN1/GluN2D
receptors relative to GluN1/GluN2A receptors. This suggests that the GluN2D-containing receptors exhibit more significant decoupling of the amino-terminal domain than GluN2A-containing
receptors. The smFRET histograms were fit into states based on Gaussian fitting guided by hidden Markov model-based analysis of the smFRET traces (Supplementary Fig. 1 and Supplementary Fig.
2). While both GluN2D- and GluN2A-containing receptors occupy similar high FRET efficiency states, GluN2D-containing receptors also populate low FRET efficiency states. This shift towards
lower FRET states results in a lower occurrence of the coupled high FRET states. In the glutamate-glycine bound state, the fraction of the high FRET conformation is ~ 10% for the
GluN1/GluN2D receptor and 65% for the GluN1/GluN2A receptor (Table 1). These fractions correlate to the Popen of less than 0.1 for GluN1/GluN2D receptors and approximately 0.5 for
GluN1/GluN2A receptors. In addition, the 10% occurrence of the super-compact conformation at the GluN2D amino-terminal domain is similar to the 6% occurrence of the low FRET state at the
pre-M1 site of GluN1/GluN2D receptors (Table 1)47. Given that decoupling at the GluN2 amino-terminal domain has been linked to channel inhibition of GluN1/GluN2A and GluN1/GluN2B receptors
and that the fraction of GluN1/GluN2D and GluN1/GluN2A receptors occupying the super-compact state correlates with their respective Popen, we can conclude that the super-compact conformation
may represent the activated state of these receptors. In addition to the conformational spread, we also investigated the conformational dynamics of the GluN2 amino-terminal domain in terms
of transition probabilities between states (Fig. 4e, f). Transition probabilities were calculated per 5 ms, as the raw smFRET data was binned to 5 ms. These data show that the GluN2D
amino-terminal domain is more dynamic relative to GluN2A in both apo and ligand-bound conditions. In particular, the low FRET states (0.46 and 0.65; Table 1), which are populated by the
GluN2D amino-terminal domain upon agonist binding, show many transitions, indicating that these conformations are highly dynamic (Fig. 4e, f). BOTTOM-UP ALLOSTERIC PATHWAY REVEALED BY
GNE-9278 While these studies illustrate the top-down allosteric pathway from the amino-terminal domain to the transmembrane segments, relatively few studies demonstrate a bottom-up
allosteric pathway52. Several positive allosteric modulators, such as GNE-9278, have been shown to bind to the GluN1 subunit pre-M1 segment. The assumption is that the mechanism of
potentiation is primarily through localized changes at the transmembrane segments39. It remains unknown if there are long-range conformational alterations. smFRET data at the GluN2
amino-terminal domain in GluN1/GluN2D in the presence of saturating 1 mM glutamate and glycine and 30 µM GNE-9278 (sample traces are shown in Fig. 5a) show a shift towards higher FRET
efficiencies, hence favoring a more coupled state of this domain (Fig. 5b). The occurrence of this coupled state is approximately 53% (Supplementary Fig. 1 and Table 1), which correlates to
the 6-fold increase in activation observed for the GluN1/GluN2D receptors in the presence of GNE-9278 (Fig. 5c). In addition, we observe a decrease in conformational dynamics, suggesting
stabilization of the coupled amino-terminal domain conformation upon GNE-9278 binding (Fig. 5d). To verify that the changes at the amino-terminal domain are not fluorophore dependent, we
performed additional smFRET experiments using Alexa 555 and Alexa 750 as the donor and acceptor fluorophores, which have an R0 of 44 Å. The smFRET histograms show similar trends as those
observed with Alexa 555 and Alexa 647, with a shift toward lower FRET upon binding glutamate and glycine relative to apo conditions (Supplementary Fig. 3a), and a shift back toward higher
FRET upon binding GNE-9278 (Supplementary Fig. 3b). These data show the robustness of the smFRET data and further confirm our results that allosteric communication between the amino-terminal
and transmembrane domains is conserved even in the modulatory mechanism of positive allosteric modulators and, more importantly, that the pathway is bidirectional. DISCUSSION The smFRET
methodology allows us to explore conformational landscapes in conjunction with protein dynamics at specific sites of interest within a protein guided by existing cryo-EM structures. In turn,
this technique allows us to correlate conformations to functional states53,54,55. Here, we utilize this methodology to study the GluN2 subunit-mediated conformational control of NMDA
receptors that correlates with functional differences between receptor subtypes. Our results reveal the occupancy of several common conformational classes between the GluN1/GluN2A and
GluN1/GluN2D receptors. However, the population shift in conformation occurrences correlates with the GluN2 subtype-specific functional properties. The similarity in the conformations
occupied at the agonist-binding domains of the GluN1/GluN2A and GluN1/GluN2D receptors suggests that the agonist-binding domains primarily act as a lever switching the receptor from resting
to active and desensitized states. The agonist-binding domains do not play a direct role in differences in receptor gating observed between the two subtypes. Furthermore, the lack of
differences between GluN1/GluN2A and GluN1/GluN2D receptors at this domain suggests that variations in glutamate and glycine affinity arise from local differences at the agonist-binding site
and not due to global cleft closure conformational changes. Such local differences in the side chains and binding site backbone were previously seen in the x-ray structures of the isolated
agonist-binding domains of the GluN2A and GluN2D subunits25,43,56. These structures did not show significant conformational differences, which is consistent with our data on the full-length
receptor. Conformational differences between GluN1/GluN2A and GluN1/GluN2D are primarily seen across the pre-M1 transmembrane segments and at the GluN2 amino-terminal domain. The GluN2
amino-terminal domain of GluN1/GluN2A can be classified into compact and super-compact conformations under agonist-bound conditions. Our data show that the GluN2 amino-terminal domain in
GluN1/GluN2D receptors also populates these states, in addition to splayed and super-splayed conformations. The GluN1/GluN2D receptors, which have a low Popen, demonstrate a shift toward the
decoupled splayed and super-splayed conformations relative to the GluN1/GluN2A receptors, which have a high Popen and populate the compact and super-compact states to a higher degree (Fig.
6). Additionally, in the presence of positive allosteric modulator GNE-9278, the GluN2 amino-terminal domain in GluN1/GluN2D receptors no longer populates the super-splayed state, resulting
in a higher occurrence of the super-compact state (Fig. 6). This suggests that the compact and super-compact conformations are pre-active and active states, while the splayed and
super-splayed conformations represent inactive states. While recent cryo-EM structures of GluN1/GluN2D show the coupled conformations (compact and super-compact), the decoupled conformations
(splayed and super-splayed) have not been observed28,29. This is most likely due to the highly dynamic nature of these decoupled states, as observed in our smFRET data, which makes them
difficult to classify. It is also interesting to note that our smFRET data measuring distances across the GluN2 amino-terminal domain differ from those recently reported for the GluN1
amino-terminal domain33. The smFRET investigations measuring distances across the GluN1 amino-terminal domain indicate that the super-compact conformation is inactive, while the compact
conformation at this domain is active. These differences indicate that the GluN1 and GluN2 subunits occupy distinct conformations. Such differences were previously observed in cryo-EM
structures of the GluN1/GluN2C receptor, which display large splaying at the GluN2 subunits and more minor conformational changes at the GluN1 subunits28,29. It is also important to note
that the smFRET measurements performed on the GluN1 subunits were acquired at 100 ms bins. A number of the transition dynamics that we observe across the GluN2D subunits are significantly
faster than this binning timescale, which would result in the averaging out of the states that we observed in our smFRET data that is binned at 5 ms. Control of receptor activation and
desensitization through inter-subunit coupling and decoupling is seen across different subtypes of the ionotropic glutamate receptor family. Our data reveal that coupling of the GluN2
amino-terminal domain is correlated with receptor activation. Similarly, coupling of the amino-terminal domains in delta receptors through stabilization by cerebellin-1 and neurexin-1β
allows these receptors to function as ligand-gated ion channels6. While coupling and decoupling occur at the amino-terminal domain in NMDA and delta receptors, these conformational changes
occur at the agonist-binding domain dimer interface in AMPA and kainate receptors. In AMPA and kainate receptors, receptor activation is reflected in the coupling of the agonist-binding
domain dimer interface, while decoupling of this interface correlates with desensitization57,58,59,60,61,62. In addition, the mechanism of extracellular domain-mediated inter-subunit
interaction controlling receptor gating is also observed in the modulation of activation by auxiliary subunits and small molecule modulators63,64,65. However, it is less understood if these
inter-subunit interactions are altered by modulators that bind near the transmembrane segments, away from the extracellular domain. Our smFRET data of GNE-9278 bound GluN1/GluN2D show that
binding of the modulator at the transmembrane segments alters the conformations and dynamics of the extracellular amino-terminal domain. Interestingly, recent smFRET and cryo-EM
investigations reveal that the allosteric inhibitor GYKI-52466, which binds in the transmembrane collar region of AMPA receptors, also produces a conformational shift in the extracellular
domain. Specifically, the binding of GYKI-52466 pushes the AMPA receptor toward occupying a decoupled inactive agonist-binding domain structure66. These findings suggest that the allosteric
communication from the pre-transmembrane region up toward the extracellular domains is conserved across different subtypes of ionotropic glutamate receptors and establishes the
bi-directionality of this allosteric pathway. METHODS DNA CONSTRUCT DESIGN All extracellular nondisulfide-bonded cysteines in wild-type _Rattus norvegicus_ GluN1 and GluN2D (NCBI accession
codes: NM017010 (GluN1), L31611 (GluN2D), D13211 (GluN2A)) were mutated to serines (C459 in N1; C231, C399, and C460 in GluN2A; C24, C245, and C485 in GluN2D). Site-directed mutagenesis was
used to further modify these cys-light constructs to create the constructs used for smFRET measurements. For the GluN1 constructs, a C-terminal Twin-Strep tag was added to GluN1 (GluN1-strep
tag), T701 and S507 were changed to cysteines (GluN1-ABD), and, lastly, F554 of GluN1 was changed to a cysteine (GluN1-TMD). The GluN2A-ABD was formed by changing Q503 and M701 to cysteine,
and the GluN2A-ATD was created by changing T174 to a cysteine. All GluN1 and the GluN2A-ABD constructs were previously used and shown to be functional by electrophysiology46,47,48,49. For
the first GluN2D construct, Q528 and M726 of GluN2D were changed to cysteines and a C-terminal His tag was introduced (GluN2D-ABD). To form the GluN2D-ATD construct, T188 was changed to a
cysteine (GluN2D-ATD). All constructs are contained in the pcDNA3.1 vector with a CMV promoter and have been shown to form functional receptors via electrophysiology (see Fig. 1). In
addition, all mutations were verified using Sanger sequencing. CELL CULTURE AND TRANSFECTION HEK293T cells (ATCC, cat. # CRL-3216, LOT 70041165) were cultured at 37 °C and 5% CO2 in growth
media containing Dulbecco’s Modified Eagle’s Medium (DMEM) (GenDepot, cat. # CM001-050) supplemented with 10% Fetal Bovine Serum (FBS) (GenDepot, cat. # F0900-050), 1 unit/mL Penicillin/ 1
ng/mL Streptomycin (P/S) (Sigma-Aldrich: P0781), and 25 mg plasmocin (Invivogen, cat. # ant-mpp). Before transfection, the cells were cultured for ~ 3–20 passages. At ~ 50–70% confluency,
the cells were transiently transfected using jetPRIME reagent (Polyplus, cat. # 101000046) with 10 µg of DNA per 10 cm dish in a 1:3 µg ratio of GluN1 to GluN2 DNA. For experiments measuring
the GluN2 ATD, cells were transfected with 2.5 µg of GluN1-strep tag and 7.5 µg of GluN2(A or D)-ATD. For experiments measuring the GluN2 ABD, cells were transfected with 2.5 µg of
GluN1-strep tag and 7.5 µg of GluN2(A or D)-ABD. For experiments measuring the GluN1 ABD, cells were transfected with 1.25 µg of GluN1-strep tag, 1.25 µg of GluN1-ABD, and 7.5 µg of GluN2(A
or D). For experiments measuring the GluN1 TMD, cells were transfected with 2.5 µg GluN1-TMD and 7.5 µg GluN2(A or D). Per the smFRET experiment, two 10 cm dishes were transfected. After
four hours of transfection, the jetPRIME reagent was replaced with growth media containing 30 µM of GluN1 antagonist DCKA (Abcam, cat. # ab120254) and 300 µM of GluN2 antagonist APV (Abcam,
cat. # ab120271) and incubated overnight. The same procedure was followed for electrophysiology experiments with the following exceptions. At 40–50% confluency, HEK293T cells in 35 mm dishes
were transfected using Lipofectamine 2000 (Invitrogen, cat. # 11668019) in optiMEM (Gibco, cat. # 11058021) and co-transfected with GFP. 30 µM of DCKA and 300 µM of APV were present during
and after transfection. ELECTROPHYSIOLOGY Whole-cell patch-clamp recordings were performed 24 h after transfection using fire-polished borosilicate glass pipettes with 3–5 MΩ resistance,
filled with internal solution (135 mM CsF, 33 mM CsOH, 2 mM MgCl2, 1 mM CaCl2, 11 mM EGTA, and 10 mM HEPES, adjusted to pH 7.4 with CsOH). The external solution (140 mM NaCl, 2.9 mM KCl, 1
mM CaCl2, and 10 mM HEPES, adjusted to pH 7.4 with NaOH) was locally applied to lifted cells using a stepper motor system (SF-77B, Warner Instruments) in the presence of 1 mM ligands
(glutamate and glycine) and in the presence or absence of 30 µM GNE-9278 (Tocris Bioscience, cat. # 6369). Recordings were performed using an Axopatch 200B amplifier (Molecular Devices) at −
60 mV holding potential, acquired at 10 kHz using pCLAMP10.7 software (Molecular Devices), and filtered online at 5 kHz. SMFRET SAMPLE PREPARATION Sample preparation was performed 24 hours
following transfection. The cells were harvested from the two 10 cm dishes. The cells were then washed in 3 mL of extracellular buffer (ECB) (135 mM NaCl, 3 mM KCl, 2 mM CaCl2, 20 mM
glucose, and 20 mM HEPES; pH 7.4). The NMDA receptors were labeled using 600 nM Alexa 555-C2-maleimide (Invitrogen, cat. # A20346) and 2.4 µM Alexa 647-C2-maleimide (Invitrogen, cat. #
A20347), and similar concentration of Alexa 555-C2-maleimide (Invitrogen) and 3.3 µM Alexa 750-C5-maleimide (Invitrogen, cat. # A30459) in 3 mL of ECB buffer for the two donor:acceptor
experiments. To allow the fluorophore dyes to bind to the cysteine residues on the NMDA receptors, the cells were incubated in the dark for 30 min at room temperature. Following incubation,
the cells were washed with ECB three times and resuspended in 2 mL of Solubilization Buffer (1% Lauryl Maltose Neopentyl Glycol (Anatrace, cat. # NG310), 2 mM Cholesterol Hydrogen Succinate
(MP Biomedicals, cat. # 101379), and protease inhibitors from Pierce Protease Inhibitor Mini Tablets (ThermoFisher Scientific, cat. # A32953) in 1X PBS). The sample was nutated at 4 °C for 1
h. Cells were then spun for 1 h at 4 °C in a Beckman Ultracentrifuge using a TLA 100.3 rotor at 100,000 × _g_. The supernatant was collected and stored on ice before use. SMFRET SLIDE
PREPARATION Bath sonication was used to clean microscope cover glasses in a solution of 5% Liquinox phosphate-free detergent (Alconox Inc., cat. # 1201-1), followed by sonication in acetone
(Thermo Scientific Chemicals, cat. # AA30698K7). The slides were incubated at 70 °C in TI-1 solution (4.3% NH4OH and 4.3% H2O2) for 5 min. The slides were washed and dried before being
subjected to plasma cleaning using a Harrick Plasma PDC-32G Plasma Cleaner. The slides were aminosilanized using a 1:40 Vectabond (Vector Laboratories: cat. # SP-1800-7) to acetone solution.
Cleaned and Vectabond-treated slides were stored under vacuum. The day before each experiment, an overnight PEG solution (0.25% w/w 5 kDa biotin-terminated PEG (NOF Corp., cat. # BP-29244)
and 25% w/w 5 kDa mPEG succinimidyl carbonate (Laysan Bio Inc., cat. # NC0788476) in 10 mM sodium bicarbonate) was applied. On the day of each experiment, the slides were treated with a
short-chain PEG solution (25 mM short-chain 333 Da MS(PEG)4 Methyl-PEG-NHS-Ester Reagent (Thermo Scientific, cat. # PI22341) in 0.1 M sodium bicarbonate) and incubated for 2 to 3 h at room
temperature. A physical chamber was constructed on top of the slides using adhesive Hybriwell chambers (Grace Bio-labs, cat. # 43018 C) and press-fit tubing connectors (Grace Bio-labs, cat.
# 460003). 36 µL of streptavidin solution (0.2 mg/mL Streptavidin (Invitrogen, cat. # S888) in 1X Imaging Buffer (1 mM nDodecyl-beta-D-maltoside (Chem-Impex Int’l Inc., cat. # 21950) and 0.2
mM Cholesteryl Hydrogen Succinate (MP Biomedicals, cat. # 1510-21-0) in 1X PBS)) was loaded into the slide chamber and allowed to incubate for 10 min at room temperature. When the molecule
of interest did not contain a Twin-Strep Tag, 10 nM of biotinylated goat Anti-Mouse IgG (H + L) secondary antibody (Jackson ImmunoResearch Laboratories, Inc., cat. # 115-065-003) was loaded
into the chamber and incubated for 20 min. The chamber was flushed using a buffer to remove unbound antibodies before applying 10 nM of anti-GluN1 mouse monoclonal primary antibody (Synaptic
Systems, cat. # 114 011). Antibody treatment was not necessary when the molecule of interest contained a genetically encoded Twin-Strep Tag. The supernatant prepared in the smFRET Sample
Preparation section above was either directly applied to the slide or diluted between 1:2 and 1:5 in 1X Imaging Buffer before application. The slides were incubated for 20 min at 4 °C. The
slide chambers were washed with reactive oxygen species (ROXS) scavenging solution (3.3% w/w glucose (Sigma-Aldrich, cat. # G8270), 3 units/mL pyranose oxidase (Sigma-Aldrich, cat. #
P4234-250UN), 0.001% w/w catalase (Sigma-Aldrich, cat. # C1345), 1 mM ascorbic acid (Sigma-Aldrich, cat. # A7506100G), and 1 mM methyl viologen (Sigma-Aldrich, cat. # 856177) in 1X imaging
Buffer, pH 7.5; plus 1 mM glutamate and/or 1 mM glycine and/or 12 μg/mL glycine oxidase (Biovision, cat. # 10835-720) and/or 30 µM GNE-9278 as needed to create the appropriate experimental
conditions). The slides were then imaged. A PicoQuant MicroTime 200 Fluorescence Lifetime Microscope and SymPhoTime 64 software were used to acquire the smFRET data. The fluorophores were
excited using 532 nm (LDH-D-TA-530; Picoquant), 637 nm (LDH-D-C-640; Picoquant), and 730 nm (LDH-D-C-730; Picoquant) lasers operated in pulsed interleaved excitation (PIE) mode with an 80
MHz pulse rate. The slides were mounted on an x-y-z piezo stage (P-733.2CD; Physik Instrumente), and single molecules were observed through an oil-immersed 100x objective. 582/64 nm and
690/70 nm emission filters were used to collect the fluorescence emission for Alexa 555 and Alexa 647, and 582/64 nm and 810/90 nm filters were used to collect the fluorescence emission for
Alexa 555 and Alexa 750. For experiments with Alexa 555 and Alexa 647, the laser intensity of 532 nm was approximately 0.8 µW, and for experiments with Alexa 555 and Alexa 750, the laser
intensity of 532 nm was approximately 5 µW. Donor and acceptor fluorophore intensities for all individual molecules were measured by the photon detection counts per 5 ms time bin, and the
corresponding FRET efficiency for each time bin was calculated. To ensure that the final traces were obtained from molecules with a single FRET pair attached, molecules were screened for
traces showing anticorrelation between the donor and acceptor intensities as well as a single photobleaching step in the donor and acceptor channels. Photoblinking events were removed from
the selected smFRET traces. The total number of molecules and total number of data points (binned to 5 ms) included in the final analysis for each condition was as follows: GluN1/GluN2D-ATD
apo = 83 molecules, 34,694 data points, glu/gly = 97 molecules, 40,095 data points, glu/gly/GNE = 80 molecules, 31,783 data points; GluN1/GluN2D-ABD apo = 105 molecules, 45,097 data points,
glu/gly = 110 molecules, 48,928 data points; GluN1-ABD/GluN2D apo = 85 molecules, 42,763 data points, glu/gly = 64 molecules, 25,366 data points; GluN1-TMDGluN2D apo = 75 molecules, 13,196
data points, glu/gly = 74 molecules, 14,242 data points; GluN1/GluN2A-ATD apo = 91 molecules, 40,309 data points, glu/gly = 81 molecules, 44,300 data points; GluN1-ABD/GluN2A apo = 135
molecules, 57,236 data points, glu/gly = 71 molecules, 23,210 data points. smFRET data for the GluN1/GluN2A -ABD, GluN1-ABD/GluN2A, and GluN1-TMD/GluN2A under apo and glu/gly conditions was
previously published46,47. For experiments measuring GluN1/GluN2D-ATD with Alexa 555 and Alexa 750, we had apo = 25 molecules, 5526 data points, glu/gly = 30 molecules, 5216 data points,
glu/gly/GNE = 31 molecules, 10,550 data points. After correcting all individual traces for background signal, cross-talk between donor and acceptor channels, differences in quantum yield,
and detector efficiency, FRET efficiency over time for each molecule was calculated using the intensity of the acceptor and donor fluorophores with the Förster equation. The individual
molecules were pooled for each measurement site and ligand condition to create cumulative histograms of the FRET efficiency distribution in MATLAB R2022b (MathWorks, Inc.). The histograms
provide a visual representation of the fractional occurrence of various smFRET efficiency states. The total number of molecules collected for final analysis was obtained from 2 to 6 days of
experiments. The mean and SEM of each measurement site and condition were determined from the data across the different days, and significance was determined by comparing the means using a
two-sided, two-sample _t_ test. Datasets with _p_-values < 0.05 are considered to be significant. Using OriginPro Version 2024 software (OriginLab Corporation), Gaussian fitting guided by
Hidden Markov model-based analysis of smFRET traces was used to reveal underlying conformational states that comprise the overall FRET efficiency distribution and transition
probabilities44,45. A summary of the preferred FRET efficiency states for all constructs and conditions alongside calculated FRET distances is shown in Table 1. A detailed protocol for
smFRET data collection and analysis has been detailed in book chapters by Litwin et al. and Paudyal et al.53,55. To determine the error associated with the orientation factor (κ), we
measured the polarization ratio for the donor (Alexa 555) and acceptor (Alexa 647) fluorophore-labeled protein for each of the constructs studied in smFRET. For these experiments, we
followed the same sample preparation steps as for smFRET experiments. Following ultracentrifugation, an antibody or affinity pull-down was performed on the supernatant. Fluorescence
polarization measurements were performed at room temperature using a PTI QuantaMaster polarization accessory. The polarization ratios were calculated from Eq. 167:
$$p=\,\frac{{I}_{{VV}}-G{I}_{{VH}}}{{I}_{{VV}}+G{I}_{{VH}}}$$ (1) Where IVV and IVH are the measured fluorescence intensities with the excitation polarizer vertically oriented and the
emission polarizer vertically and horizontally oriented, respectively. G is the grating correction factor for the detection system. As polarization ratios did not satisfy the
Anderson-Darling normality test, they were compared using non-parametric Kruskal-Wallis and Dunn’s multiple comparisons tests, which were performed using GraphPad Prism version 10.0.3
(GraphPad Software). _P_-values < 0.05 are considered significant. The calculated polarization ratio values are provided in Supplementary Table 1. The values range from 0.07 to 0.26, and
there are no significant changes in the polarization ratios between the conditions measured (apo, liganded, and modulator bound) for a given site. Thus, it is unlikely that the changes in
smFRET between the different conditions (apo, liganded, and modulator bound) measured here for a given site arise due to differences in the orientation factor68. To ensure that our data
acquisition and analysis were not biased, one set of data for the amino-terminal domain smFRET was obtained blind for each investigated condition. The samples were prepared and unidentified
by one lab member and collected and analyzed by a second without knowledge of the conditions. We observed that this set was statistically similar to the data obtained without blinding.
REPORTING SUMMARY Further information on research design is available in the Nature Portfolio Reporting Summary linked to this article. DATA AVAILABILITY The data that support this study are
available from the corresponding authors upon request. Source data are provided in this paper. The structural data referred to within this study was previously published and can be accessed
via the Protein Data Bank (PDB) under the following accession numbers 7EOS, 8E96. Source data are provided in this paper. REFERENCES * Iacobucci, G. J. & Popescu, G. K. NMDA receptors:
linking physiological output to biophysical operation. _Nat. Rev. Neurosci._ 18, 236–249 (2017). Article PubMed PubMed Central CAS Google Scholar * Hansen, K. B., Yi, F., Perszyk, R.
E., Menniti, F. S. & Traynelis, S. F. NMDA Receptors in the Central Nervous System. _Methods Mol. Biol._ 1677, 1–80 (2017). Article PubMed PubMed Central CAS Google Scholar *
Traynelis, S. F. et al. Glutamate receptor ion channels: structure, regulation, and function. _Pharmacol. Rev._ 62, 405–496 (2010). Article PubMed PubMed Central CAS Google Scholar *
Hollmann, M. & Heinemann, S. Cloned glutamate receptors. _Annu. Rev. Neurosci._ 17, 31–108 (1994). Article PubMed CAS Google Scholar * Hansen, K. B. et al. Structure, function, and
pharmacology of glutamate receptor ion channels. _Pharmacol. Rev._ 73, 298–487 (2021). Article PubMed PubMed Central Google Scholar * Carrillo, E., Gonzalez, C. U., Berka, V. &
Jayaraman, V. Delta glutamate receptors are functional glycine- and D-serine-gated cation channels in situ. _Sci. Adv._ 7, eabk2200 (2021). Article ADS PubMed PubMed Central CAS Google
Scholar * Newcomer, J. W., Farber, N. B. & Olney, J. W. NMDA receptor function, memory, and brain aging. _Dialogues Clin. Neurosci._ 2, 219–232 (2000). Article PubMed PubMed Central
CAS Google Scholar * Dupuis, J. P., Nicole, O. & Groc, L. NMDA receptor functions in health and disease: Old actor, new dimensions. _Neuron_ 111, 2312–2328 (2023). Article PubMed CAS
Google Scholar * Iacobucci, G. J. et al. Complex functional phenotypes of NMDA receptor disease variants. _Mol. Psychiatry_ 27, 5113–5123 (2022). Article PubMed CAS Google Scholar *
Kleckner, N. W. & Dingledine, R. Requirement for glycine in activation of NMDA-receptors expressed in Xenopus oocytes. _Science_ 241, 835–837 (1988). Article ADS PubMed CAS Google
Scholar * Benveniste, M. & Mayer, M. L. Kinetic analysis of antagonist action at N-methyl-D-aspartic acid receptors. Two binding sites each for glutamate and glycine. _Biophys. J._ 59,
560–573 (1991). Article ADS PubMed PubMed Central CAS Google Scholar * Lee, C. H. et al. NMDA receptor structures reveal subunit arrangement and pore architecture. _Nature_ 511,
191–197 (2014). Article ADS PubMed PubMed Central CAS Google Scholar * Karakas, E. & Furukawa, H. Crystal structure of a heterotetrameric NMDA receptor ion channel. _Science_ 344,
992–997 (2014). Article ADS PubMed PubMed Central CAS Google Scholar * Clements, J. D. & Westbrook, G. L. Activation kinetics reveal the number of glutamate and glycine binding
sites on the N-methyl-D-aspartate receptor. _Neuron_ 7, 605–613 (1991). Article PubMed CAS Google Scholar * Pérez-Otaño, I., Larsen, R. S. & Wesseling, J. F. Emerging roles of
GluN3-containing NMDA receptors in the CNS. _Nat. Rev. Neurosci._ 17, 623–635 (2016). Article PubMed Google Scholar * Reiner, A. & Levitz, J. Glutamatergic signaling in the central
nervous system: Ionotropic and metabotropic receptors in concert. _Neuron_ 98, 1080–1098 (2018). Article PubMed PubMed Central CAS Google Scholar * Paoletti, P., Bellone, C. & Zhou,
Q. NMDA receptor subunit diversity: impact on receptor properties, synaptic plasticity and disease. _Nat. Rev. Neurosci._ 14, 383–400 (2013). Article PubMed CAS Google Scholar *
Erreger, K., Dravid, S. M., Banke, T. G., Wyllie, D. J. & Traynelis, S. F. Subunit-specific gating controls rat NR1/NR2A and NR1/NR2B NMDA channel kinetics and synaptic signalling
profiles. _J. Physiol._ 563, 345–358 (2005). Article PubMed PubMed Central CAS Google Scholar * Cull-Candy, S., Brickley, S. & Farrant, M. NMDA receptor subunits: diversity,
development and disease. _Curr. Opin. Neurobiol._ 11, 327–335 (2001). Article PubMed CAS Google Scholar * Vicini, S. et al. Functional and pharmacological differences between recombinant
N-methyl-D-aspartate receptors. _J. Neurophysiol._ 79, 555–566 (1998). Article PubMed CAS Google Scholar * Monyer, H. et al. Heteromeric NMDA receptors: molecular and functional
distinction of subtypes. _Science_ 256, 1217–1221 (1992). Article ADS PubMed CAS Google Scholar * Yuan, H., Hansen, K. B., Vance, K. M., Ogden, K. K. & Traynelis, S. F. Control of
NMDA receptor function by the NR2 subunit amino-terminal domain. _J. Neurosci._ 29, 12045–12058 (2009). Article PubMed PubMed Central CAS Google Scholar * Hansen, K. B. et al.
Structure, function, and allosteric modulation of NMDA receptors. _J. Gen. Physiol._ 150, 1081–1105 (2018). Article ADS PubMed PubMed Central CAS Google Scholar * Misra, C., Brickley,
S. G., Wyllie, D. J. & Cull-Candy, S. G. Slow deactivation kinetics of NMDA receptors containing NR1 and NR2D subunits in rat cerebellar Purkinje cells. _J. Physiol._ 525, 299–305
(2000). Article PubMed PubMed Central CAS Google Scholar * Vance, K. M., Simorowski, N., Traynelis, S. F. & Furukawa, H. Ligand-specific deactivation time course of GluN1/GluN2D
NMDA receptors. _Nat. Commun._ 2, 294 (2011). Article ADS PubMed Google Scholar * Chen, P. E. et al. Modulation of glycine potency in rat recombinant NMDA receptors containing chimeric
NR2A/2D subunits expressed in Xenopus laevis oocytes. _J. Physiol._ 586, 227–245 (2008). Article PubMed CAS Google Scholar * Hansen, K. B. et al. Structural determinants of agonist
efficacy at the glutamate binding site of N-methyl-D-aspartate receptors. _Mol. Pharmacol._ 84, 114–127 (2013). Article PubMed PubMed Central CAS Google Scholar * Chou, T. H., Kang, H.,
Simorowski, N., Traynelis, S. F. & Furukawa, H. Structural insights into assembly and function of GluN1-2C, GluN1-2A-2C, and GluN1-2D NMDARs. _Mol. Cell_ 82, 4548–4563 (2022). Article
PubMed PubMed Central CAS Google Scholar * Zhang, J. et al. Distinct structure and gating mechanism in diverse NMDA receptors with GluN2C and GluN2D subunits. _Nat. Struct. Mol. Biol._
30, 629–639 (2023). Article PubMed CAS Google Scholar * Wang, H. et al. Gating mechanism and a modulatory niche of human GluN1-GluN2A NMDA receptors. _Neuron_ 109, 2443–2456 (2021).
Article PubMed CAS Google Scholar * Jalali-Yazdi, F., Chowdhury, S., Yoshioka, C. & Gouaux, E. Mechanisms for Zinc and Proton Inhibition of the GluN1/GluN2A NMDA Receptor. _Cell_
175, 1520–1532 (2018). Article PubMed PubMed Central CAS Google Scholar * Zhang, J. B. et al. Structural basis of the proton sensitivity of human GluN1-GluN2A NMDA receptors. _Cell
Rep._ 25, 3582–3590 (2018). Article PubMed CAS Google Scholar * Bleier, J. et al. Subtype-specific conformational landscape of NMDA receptor gating. _Cell Rep._ 43, 114634 (2024).
Article PubMed PubMed Central CAS Google Scholar * Madry, C., Mesic, I., Betz, H. & Laube, B. The N-terminal domains of both NR1 and NR2 subunits determine allosteric Zn2+
inhibition and glycine affinity of N-methyl-d-aspartate receptors. _Mol. Pharmacol._ 72, 1535–1544 (2007). Article PubMed CAS Google Scholar * Tajima, N. et al. Activation of NMDA
receptors and the mechanism of inhibition by ifenprodil. _Nature_ 534, 63–68 (2016). Article ADS PubMed PubMed Central CAS Google Scholar * Tian, M. & Ye, S. Allosteric regulation
in NMDA receptors revealed by the genetically encoded photo-cross-linkers. _Sci. Rep._ 6, 34751 (2016). Article ADS PubMed PubMed Central CAS Google Scholar * Hackos, D. H. &
Hanson, J. E. Diverse modes of NMDA receptor positive allosteric modulation: Mechanisms and consequences. _Neuropharmacology_ 112, 34–45 (2017). Article PubMed CAS Google Scholar *
Geoffroy, C., Paoletti, P. & Mony, L. Positive allosteric modulation of NMDA receptors: mechanisms, physiological impact and therapeutic potential. _J. Physiol._ 600, 233–259 (2022).
Article PubMed CAS Google Scholar * Wang, T. M. et al. A novel NMDA receptor positive allosteric modulator that acts via the transmembrane domain. _Neuropharmacology_ 121, 204–218
(2017). Article PubMed CAS Google Scholar * Perszyk, R. E. et al. Biased modulators of NMDA receptors control channel opening and ion selectivity. _Nat. Chem. Biol._ 16, 188–196 (2020).
Article PubMed PubMed Central CAS Google Scholar * Sirrieh, R. E., MacLean, D. M. & Jayaraman, V. A conserved structural mechanism of NMDA receptor inhibition: A comparison of
ifenprodil and zinc. _J. Gen. Physiol._ 146, 173–181 (2015). Article PubMed PubMed Central CAS Google Scholar * Sirrieh, R. E., MacLean, D. M. & Jayaraman, V. Amino-terminal domain
tetramer organization and structural effects of zinc binding in the N-methyl-D-aspartate (NMDA) receptor. _J. Biol. Chem._ 288, 22555–22564 (2013). Article PubMed PubMed Central CAS
Google Scholar * Hackos, D. H. et al. Positive allosteric modulators of gluN2A-containing NMDARs with distinct modes of action and impacts on circuit function. _Neuron_ 89, 983–999 (2016).
Article PubMed CAS Google Scholar * McKinney, S. A., Joo, C. & Ha, T. Analysis of single-molecule FRET trajectories using hidden Markov modeling. _Biophys. J._ 91, 1941–1951 (2006).
Article ADS PubMed PubMed Central CAS Google Scholar * Liu, Y., Park, J., Dahmen, K. A., Chemla, Y. R. & Ha, T. A comparative study of multivariate and univariate hidden Markov
modelings in time-binned single-molecule FRET data analysis. _J. Phys. Chem. B_ 114, 5386–5403 (2010). Article PubMed CAS Google Scholar * Durham, R. J. et al. Conformational spread and
dynamics in allostery of NMDA receptors. _Proc. Natl. Acad. Sci. USA_ 117, 3839–3847 (2020). Article ADS PubMed PubMed Central CAS Google Scholar * Dolino, D. M. et al. The
structure-energy landscape of NMDA receptor gating. _Nat. Chem. Biol._ 13, 1232–1238 (2017). Article PubMed PubMed Central CAS Google Scholar * Dolino, D. M. et al. Structural dynamics
of the glycine-binding domain of the N-Methyl-D-aspartate receptor. _J. Biol. Chem._ 290, 797–804 (2015). Article PubMed CAS Google Scholar * Dolino, D. M., Rezaei Adariani, S., Shaikh,
S. A., Jayaraman, V. & Sanabria, H. Conformational selection and submillisecond dynamics of the ligand-binding domain of the N-methyl-d-aspartate receptor. _J. Biol. Chem._ 291,
16175–16185 (2016). Article PubMed PubMed Central CAS Google Scholar * Amico-Ruvio S. A. et al. Contributions by N-terminal domains to NMDA receptor currents. Preprint at _bioRxiv_
https://doi.org/10.1101/2020.08.21.261388 (2020). * Zhu, S. et al. Mechanism of NMDA receptor inhibition and activation. _Cell_ 165, 704–714 (2016). Article PubMed PubMed Central CAS
Google Scholar * Bhatia, N. K., Carrillo, E., Durham, R. J., Berka, V. & Jayaraman, V. Allosteric changes in the NMDA receptor associated with calcium-dependent inactivation. _Biophys.
J._ 119, 2349–2359 (2020). Article ADS PubMed PubMed Central CAS Google Scholar * Paudyal, N., Bhatia, N. K. & Jayaraman, V. Single molecule FRET methodology for investigating
glutamate receptors. _Methods Enzymol._ 652, 193–212 (2021). Article PubMed PubMed Central CAS Google Scholar * Durham, R. J., Latham, D. R., Sanabria, H. & Jayaraman, V. Structural
dynamics of glutamate signaling systems by smFRET. _Biophys. J._ 119, 1929–1936 (2020). Article ADS PubMed PubMed Central CAS Google Scholar * Litwin, D. B., Durham, R. J. &
Jayaraman, V. Single-molecule FRET methods to study glutamate receptors. _Methods Mol. Biol._ 1941, 3–16 (2019). Article PubMed PubMed Central CAS Google Scholar * Yi, F. et al.
Structural basis for negative allosteric modulation of GluN2A-containing NMDA receptors. _Neuron_ 91, 1316–1329 (2016). Article PubMed PubMed Central CAS Google Scholar * Armstrong, N.
& Gouaux, E. Mechanisms for activation and antagonism of an AMPA-sensitive glutamate receptor crystal structures of the GluR2 ligand binding core. _Neuron_ 28, 165–181 (2000). Article
PubMed CAS Google Scholar * Dürr, K. L. et al. Structure and dynamics of AMPA receptor GluA2 in resting, pre-open, and desensitized states. _Cell_ 158, 778–792 (2014). Article PubMed
PubMed Central Google Scholar * Prieto, M. L. & Wollmuth, L. P. Gating modes in AMPA receptors. _J. Neurosci._ 30, 4449–4459 (2010). Article PubMed PubMed Central CAS Google
Scholar * Du, M., Rambhadran, A. & Jayaraman, V. Luminescence resonance energy transfer investigation of conformational changes in the ligand binding domain of a kainate receptor. _J.
Biol. Chem._ 283, 27074–27078 (2008). Article PubMed PubMed Central CAS Google Scholar * Litwin, D. B., Carrillo, E., Shaikh, S. A., Berka, V. & Jayaraman, V. The structural
arrangement at intersubunit interfaces in homomeric kainate receptors. _Sci. Rep._ 9, 6969 (2019). Article ADS PubMed PubMed Central Google Scholar * Litwin, D. B., Paudyal, N.,
Carrillo, E., Berka, V. & Jayaraman, V. The structural arrangement and dynamics of the heteromeric GluK2/GluK5 kainate receptor as determined by smFRET. _Biochim. Biophys. Acta
Biomembr._ 1862, 183001 (2020). Article PubMed CAS Google Scholar * Carrillo E. et al. Mechanism of modulation of AMPA receptors by TARP-γ8. _J. Gen. Physiol._ 152, (2020). * Chen, S. et
al. Activation and desensitization mechanism of AMPA receptor-TARP complex by cryo-EM. _Cell_ 170, 1234–1246 (2017). Article PubMed PubMed Central CAS Google Scholar * Paudyal, N., Das
A., Carrillo, E., Berka, V. & Jayaraman, V. Partial agonism in heteromeric GLUK2/GLUK5 kainate receptor. _Proteins_ https://doi.org/10.1002/prot.26565 (2023). * Hale, W. D. et al.
Allosteric competition and inhibition in AMPA receptors. _Nat. Struct. Mol. Biol_. (2024). * Lakowicz J. R. _Principles of Fluorescence Spectroscopy_, 3rd edn, (2006). * Haas, E.,
Katchalski-Katzir, E. & Steinberg, I. Z. Effect of the orientation of donor and acceptor on the probability of energy transfer involving electronic transitions of mixed polarization.
_Biochemistry_ 17, 5064–5070 (1978). Article PubMed CAS Google Scholar Download references ACKNOWLEDGEMENTS This project was supported by a training fellowship from the Gulf Coast
Consortia on the Houston Area Molecular Biophysics Program (Grant No. T32 GM008280) to P.B. and National Institutes of Health Grant (R35GM122528) to V.J. The authors declare no competing
financial interests. AUTHOR INFORMATION AUTHORS AND AFFILIATIONS * The University of Texas MD Anderson Cancer Center UTHealth Houston Graduate School of Biomedical Sciences, Houston, TX, USA
Paula A. Bender & Vasanthi Jayaraman * Department of Biochemistry and Molecular Biology, Center for Membrane Biology, University of Texas Health Science Center at Houston, Houston, TX,
USA Paula A. Bender, Subhajit Chakraborty, Ryan J. Durham, Vladimir Berka, Elisa Carrillo & Vasanthi Jayaraman Authors * Paula A. Bender View author publications You can also search for
this author inPubMed Google Scholar * Subhajit Chakraborty View author publications You can also search for this author inPubMed Google Scholar * Ryan J. Durham View author publications You
can also search for this author inPubMed Google Scholar * Vladimir Berka View author publications You can also search for this author inPubMed Google Scholar * Elisa Carrillo View author
publications You can also search for this author inPubMed Google Scholar * Vasanthi Jayaraman View author publications You can also search for this author inPubMed Google Scholar
CONTRIBUTIONS P.A.B. performed the smFRET measurements, analyzed the smFRET data, and wrote and edited the manuscript. E.C. performed the electrophysiology measurements analyzed the
electrophysiology data, and also assisted with cell culture. S.C. performed part of the smFRET experiments for GluN2D, and for GluN2D with GNE-9278, R.J.D. performed part of the smFRET
experiments for GluN2D, and V.B. trained P.A.B. to do the smFRET experiments and performed part of the smFRET experiments and analyzed the smFRET data. V.J. designed the research, analyzed
the data, and wrote and edited the manuscript. CORRESPONDING AUTHOR Correspondence to Vasanthi Jayaraman. ETHICS DECLARATIONS COMPETING INTERESTS The authors declare they have no competing
interests. PEER REVIEW PEER REVIEW INFORMATION _Nature Communications_ thanks Paul Selvin, who co-reviewed with Rohit Vaidya, and the other anonymous reviewers for their contribution to the
peer review of this work. A peer review file is available. ADDITIONAL INFORMATION PUBLISHER’S NOTE Springer Nature remains neutral with regard to jurisdictional claims in published maps and
institutional affiliations. SUPPLEMENTARY INFORMATION SUPPLEMENTARY INFORMATION REPORTING SUMMARY TRANSPARENT PEER REVIEW FILE SOURCE DATA SOURCE DATA RIGHTS AND PERMISSIONS OPEN ACCESS This
article is licensed under a Creative Commons Attribution-NonCommercial-NoDerivatives 4.0 International License, which permits any non-commercial use, sharing, distribution and reproduction
in any medium or format, as long as you give appropriate credit to the original author(s) and the source, provide a link to the Creative Commons licence, and indicate if you modified the
licensed material. You do not have permission under this licence to share adapted material derived from this article or parts of it. The images or other third party material in this article
are included in the article’s Creative Commons licence, unless indicated otherwise in a credit line to the material. If material is not included in the article’s Creative Commons licence and
your intended use is not permitted by statutory regulation or exceeds the permitted use, you will need to obtain permission directly from the copyright holder. To view a copy of this
licence, visit http://creativecommons.org/licenses/by-nc-nd/4.0/. Reprints and permissions ABOUT THIS ARTICLE CITE THIS ARTICLE Bender, P.A., Chakraborty, S., Durham, R.J. _et al._
Bi-directional allosteric pathway in NMDA receptor activation and modulation. _Nat Commun_ 15, 8841 (2024). https://doi.org/10.1038/s41467-024-53181-w Download citation * Received: 16 April
2024 * Accepted: 04 October 2024 * Published: 13 October 2024 * DOI: https://doi.org/10.1038/s41467-024-53181-w SHARE THIS ARTICLE Anyone you share the following link with will be able to
read this content: Get shareable link Sorry, a shareable link is not currently available for this article. Copy to clipboard Provided by the Springer Nature SharedIt content-sharing
initiative