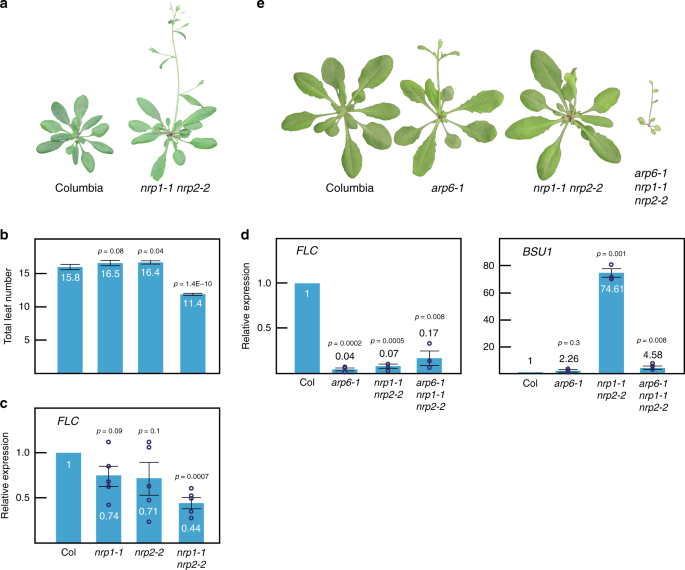
- Select a language for the TTS:
- UK English Female
- UK English Male
- US English Female
- US English Male
- Australian Female
- Australian Male
- Language selected: (auto detect) - EN
Play all audios:
ABSTRACT In eukaryotes, DNA wraps around histones to form nucleosomes, which are compacted into chromatin. DNA-templated processes, including transcription, require chromatin disassembly and
reassembly mediated by histone chaperones. Additionally, distinct histone variants can replace core histones to regulate chromatin structure and function. Although replacement of H2A with
the evolutionarily conserved H2A.Z via the SWR1 histone chaperone complex has been extensively studied, in plants little is known about how a reduction of H2A.Z levels can be achieved. Here,
we show that NRP proteins cause a decrease of H2A.Z-containing nucleosomes in Arabidopsis under standard growing conditions. _nrp1-1 nrp2-2_ double mutants show an over-accumulation of
H2A.Z genome-wide, especially at heterochromatic regions normally H2A.Z-depleted in wild-type plants. Our work suggests that NRP proteins regulate gene expression by counteracting SWR1,
thereby preventing excessive accumulation of H2A.Z. SIMILAR CONTENT BEING VIEWED BY OTHERS HISTONE CHAPERONE ASF1 MEDIATES H3.3-H4 DEPOSITION IN ARABIDOPSIS Article Open access 15 November
2022 NUA4 AND H2A.Z CONTROL ENVIRONMENTAL RESPONSES AND AUTOTROPHIC GROWTH IN _ARABIDOPSIS_ Article Open access 12 January 2022 NODULIN HOMEOBOX IS REQUIRED FOR HETEROCHROMATIN HOMEOSTASIS
IN _ARABIDOPSIS_ Article Open access 27 August 2022 INTRODUCTION DNA encodes the genetic information in the cell, but in all eukaryotes, DNA is rarely found naked but usually packaged into a
higher-order structure termed chromatin, which is composed of DNA and histones. The basic unit of the chromatin is the nucleosome, consisting of 147 base pairs of DNA wrapped around the
octamer, which is formed by one Histone3—Histone4 tetramer (H3-H4)x2 and two Histone2 dimers (H2A-H2B)x21. Canonical histones can be replaced by histone variants in a replication-independent
manner. Among H2A variants, H2A.Z is one of the most evolutionary conserved2, and has important implications not only in the regulation of gene expression but also in genome stability, cell
cycle, DNA repair, recombination, female meiosis, response to high temperatures as well as different kinds of biotic and abiotic stresses3,4,5,6,7,8,9,10,11. H2A.Z is typically found in the
arms of the chromosomes and mainly excluded from chromocenters12. In particular, H2A.Z is usually enriched at the first nucleosome after the transcriptional start site (+1 nucleosome),
although it can be found at a lower level in gene bodies13. Regarding gene regulation, it has been proposed that the presence of H2A.Z at the +1 nucleosome is associated with transcriptional
activity, whereas H2A.Z has a repressive effect on transcription when located across gene bodies8. In addition, a recent report proposes that the effect of H2A.Z on transcription depends on
the post-translational modification it may bear, where monoubiquitylation of H2A.Z would have a repressive effect on transcriptional activity while acetylated H2A.Z would serve as an
activating mark14. H2A.Z is deposited by the Swi/Snf2 Related1 (SWR1) complex, which is a chromatin remodeler that specifically replaces the H2A-H2B dimer with the H2A.Z-H2B dimer in a
stepwise reaction that requires ATP15,16. It is still uncertain what is the precise mechanism by which SWR1 is recruited to the chromatin. However, in yeast, acetylation of H4 located at
promoters is required for the proper deposition of H2A.Z at the +1 nucleosome17,18,19. Related to that, two recent reports suggest that METHYL-CpG-BINDING DOMAIN9 may guide SWR1 to open
chromatin regions nearby active genes in Arabidopsis20,21. Nucleosome assembly protein 1 (NAP1) was first described in _Xenopus leavis_ as an H2A/H2B histone chaperone that promotes
nucleosome assembly in vitro22. Subsequently, NAP1 was shown to be involved in H2A/H2B trafficking and to facilitate nucleosome disassembly23,24. NAP1 is evolutionarily conserved from yeast
to humans. In Arabidopsis, the NAP1 family consists of six members with similarity to the yeast H2A/H2B histone chaperone NAP1 and human SET/TAF-Iβ25: NAP1;1, NAP1;2, NAP1;3, NAP1;4, as well
as the two closely related orthologues NAP1-RELATED PROTEIN 1 (NRP1) and NRP2. Interestingly, NRP1 and 2 are the two proteins that have diverged the most from the founding member AtNAP126,
which raises the possibility of some degree of functional diversity. In Arabidopsis, NRP proteins have been implicated in several biological processes, including cell-cycle control, root
meristem formation, heat tolerance, DNA repair, somatic homologous recombination, and genome defense under genotoxic stress25,27,28,29. NRP proteins are localized mainly in the nucleus and
bind H2A, H2B, H3, and H4 histones25,30. However, a molecular mechanism for these proteins has not been clearly established. Here, we show that NRP proteins genetically interact with the
core components of SWR1 and associate with H2A.Z in vivo. We have also found that in _nrp1-1 nrp2-2_, H2A.Z shows an altered pattern and excessive levels compared to wild-type, a phenotype
that is consistent with NRP1 localization. Together these data suggest that NRP proteins counteract the activity of the SWR1 complex and contribute to the dynamic regulation of H2A.Z in
Arabidopsis. RESULTS NRPS REGULATE THE EXPRESSION OF DEVELOPMENTAL KEY GENES The _nrp1-1 nrp2-1_ double mutant shows a root developmental defect as the only reported apparent morphological
phenotype28. The _nrp2-1_ mutant carries a T-DNA insertion in a non-coding region28, but in this study, we have used _nrp__2-2_ allele instead, which carries a T-DNA insertion in the coding
region and therefore it is likely a null allele. We found that _nrp1-1_ and _nrp2-2_ single mutants did not display any obvious morphological phenotype. However, the _nrp1-1 nrp2-2_ double
mutant showed a slightly early flowering phenotype that correlated with lower levels of _FLOWERING LOCUS C_ (_FLC_) expression compared to wild-type (Fig. 1a–c). To find additional genes
whose expression was affected by mutations in _NRP_ genes, we performed RNA-Seq in Columbia, _nrp1-1_, _nrp2-2_, and the _nrp1-1 nrp2-2_ double mutant. Among the misregulated genes, we found
that _BRI1 SUPPRESSOR 1_ (_BSU1_) transcript levels were dramatically increased in _nrp1-1 nrp2-2_ double mutants relative to wild-type plants, which was in contrast with previous
transcriptomic analyses using _nrp1-1 nrp2-1_28 (Fig. 1d). The phosphatase BSU1 dephosphorylates the kinase BRASSINOSTEROID INSENSITIVE2 (BIN2), which positively regulates the
brassinosteroid signaling pathway31. Indeed, simultaneous disruption of _NRP1_ and _2_ suppressed phenotypes arising from _BIN2_ overexpression, likely due to BSU1-mediated dephosphorylation
of BIN2, since BIN2 protein levels were unaltered (Supplementary Fig. 1a, b). The kinase BRASSINOSTEROIDS INSENSITIVE1 (=BRI1) activates BSU132. The weak mutant allele _bri1-119_, displays
a pleiotropic phenotype including infertility, small dark green rosettes, inward curled leaves, and extra axillary inflorescences33, which was alleviated in the _nrp1-1 nrp2-2_ background
(Supplementary Fig. 1a), further supporting the overexpression of _BSU1_ upon loss of NRP proteins. THE SWR1 COMPLEX IS REQUIRED FOR _NRP1-1 NRP2-2_ PHENOTYPE To determine how NRP proteins
regulate the expression of these genes, we examined genetic interactions with other histone chaperones. Specifically, we crossed the _nrp1-1 nrp2-2_ double mutant with mutants encoding
putative H2A-H2B chaperones. We found that _nrp1-1 nrp2-2_ double mutants enhanced _arp6-1_ phenotype when we looked at the overall morphological phenotype (Fig. 1e). Unexpectedly,
transcript levels of _BSU1_ were restored to nearly wild-type levels in the _arp6-1 nrp1-1 nrp2-2_ triple mutant compared to the _nrp1-1 nrp2-2_ double mutant. We did not observe a full
restoration of the expression of _FLC_, but only a slight recovery (Fig. 1d). These data suggest that ACTIN RELATED PROTEIN6 (ARP6) is required for the altered expression of these genes in
the _nrp1-1 nrp2-2_ mutant background. ARP6 is a core component protein of the Arabidopsis SWR1 complex, which replaces H2A-H2B by H2A.Z-H2B in an ATP-dependent manner16. We hypothesized
that SWR1 activity could be essential to explain the observed phenotypes. Indeed, mutants affecting other known components of the SWR1 complex, SERRATED LEAVES AND EARLY FLOWERING (SEF) and
PHOTOPERIOD-INDEPENDENT EARLY FLOWERING1 (PIE1), when crossed to the _nrp1-1 nrp2-2_ double mutant, yielded similar results (Supplementary Fig. 2). Also, combining the _nrp1-1 nrp2-2_ double
mutant with mutations in _HTA9_ and _HTA11_, two of the three genes encoding H2A.Z in Arabidopsis, suppressed the up-regulation of _BSU1_ in the _nrp1-1 nrp2-2_ double mutant (Supplementary
Fig. 2). Thus, H2A.Z is required for the increased expression of _BSU1_ in the _nrp1-1 nrp2-2_ background. We observed a much more severe phenotype when mutations in _NRP1_ and _2_ were
combined with SWR1 core components compared to mutations in H2A.Z coding genes themselves (Supplementary Fig. 2). This might be due to the involvement of SWR1 complex components in cellular
mechanisms other than H2A.Z deposition or the presence of wild-type HTA8 in the _hta9-1 hta11-1_ double mutant. NRP PROTEINS INTERACT WITH H2A.Z IN VIVO To further illuminate the molecular
function of NRP proteins, we constructed Myc- and Flag-tagged versions of _NRP1_ and _2_ driven by their native 5′ regulatory regions for complementation analysis. We found that the _BSU1_
overexpression phenotype of the _nrp1-1 nrp2-2_ double mutant was complemented by the expression of N-terminally tagged 9xMyc-NRP1, as well as C-terminally, tagged NRP2-9xMyc, and
NRP2-3xFlag (Supplementary Fig. 3). We subsequently performed experiments with these complementing constructs in their respective single mutant background. We used both _NRP2-3xFlag_ and
_NRP2-9xMyc_ complementing lines for immunoprecipitation, followed by Mass Spectrometry. We found that NRP1 was present in all replicates in a nearly 1:1 ratio with NRP2, which is consistent
with these two proteins forming heteromers in vivo34 (Table 1). We confirmed this interaction by co-immunoprecipitation assays in F1 lines expressing both NRP2-3xFlag and 9xMyc-NRP1
(Supplementary Fig. 4). Given that NRP proteins share some degree of similarity with NAP1 and genetically interact with core components of Arabidopsis SWR1, we next tested whether they
interact with either H2A or H2A.Z in vivo. Previous works showed that NRP1 could bind with H2A, H2B and H3 in pull-down assays28 as well as H2B, H3, H4, H2A-H2B, and H3-H4 tetramers as
measured by ITC30. Consistently, co-IP assays confirmed that NRP1 and 2 physically interact with canonical H2A. In Addition, we found a strong interaction with the replacement variant H2A.Z
in vivo (Fig. 2a–c). A recent study14 showed that H2A.Z can be monoubiquitinated in Arabidopsis; co-IP assays showed that both NRP proteins bind unmodified as well as monoubiquitinated H2A.Z
in vivo (Fig. 2d). _NRP1-1 NRP2-2_ SHOWS OVERACCUMULATION OF H2A.Z Given our findings that disruption of genes encoding H2A.Z or the SWR1 complex suppresses the phenotype of _nrp1-1 nrp2-2_
at _BSU1_, and that NRP proteins can bind not only H2A but also H2A.Z, we hypothesized that NRP proteins might act to reduce H2A.Z locally. Western blot analysis suggested that global
levels of H2A and H2A.Z were comparable in _nrp1-1 nrp2-2_ and wild-type plants (Supplementary Fig. 5). To investigate changes in the levels of H2A.Z in _nrp1-1 nrp2-2_ genome-wide, we
performed H2A.Z Chromatin Immunoprecipitation followed by whole-genome sequencing (ChIP-Seq). First, we examined H2A.Z peaks defined in wild-type (_n_ = 21,024) and observed significantly
higher average occupancies of H2A.Z in the _nrp1-1 nrp2-2_ double mutant compared to wild-type (Fig. 3a). Moreover, metagene analysis also showed a general accumulation of H2A.Z in the
double mutant, except in regions where H2A.Z occupancy is naturally low, such as within and immediately upstream of the transcription start sites and transcription termination sites (TSS and
TTS) (Fig. 3b). Additionally, we found 1753 highly significant H2A.Z peaks that were dependent on _nrp1-1 nrp2-2_ (_p_-value < 2.2e-16) (Fig. 3c). These peaks fall mainly in regions
annotated as coding genes (1467 coding / 286 TEs Vs 1334 coding / 419 TEs that would be expected from a randomly shuffled control). Also, consistent with our genetic data, H2A.Z occupancy in
the _arp6-1 nrp1-1 nrp2-2_ triple mutant was slightly higher than that in the _arp6-1_ single mutant (Fig. 3c and d). We validated H2A.Z ChIP-Seq bioinformatic analyses by ChIP-qPCR at
selected loci (Supplementary Fig. 6). The increased occupancy of H2A.Z observed in _nrp1-1 nrp2-2_ occurred predominantly at lowly expressed genes (Fig. 3e), which is consistent with the
fact that lowly expressed genes typically show high levels of H2A.Z14,35. Next, we performed ChIP-qPCR to assess the occupancy of H2A.Z at two putative NRP1/2 targets; _FLC_ and _BSU1_ (Fig.
3f). The analysis showed that compared to wild-type, _nrp1-1 nrp2-2_ double mutant displayed higher occupancy of H2A.Z at both loci, within regions that were previously defined as H2A.Z
peaks13 (Fig. 3g and Supplementary Fig. 7). However, we did not observe any significant enrichment in low abundance H2A.Z regions nor the negative control _ACTIN2_ (Fig. 3g). These data
suggest that NRP proteins might mitigate excessive deposition of H2A.Z. SWR1 AND NRPS HAVE OPPOSITE EFFECTS ON TRANSCRIPTION Since it has been established that low levels of H2A.Z at the
first intron of _FLC_ are correlated with a reduction in its expression12,36, it was striking that we detected higher H2A.Z levels in the first intron of _FLC_ in _nrp1-1 nrp2-2_ compared to
wild-type and observed a reduced expression of _FLC_ (Fig. 3f, g, and Supplementary Fig. 7). We searched for a possible explanation and found that in _nrp1-1 nrp2-2_ double mutant chromatin
in the first intron of _FLC_ appears to be denser compared to wild-type. Although we do not know exactly the precise mechanism, it is possible that compact chromatin around _FLC_ regulatory
region might have a negative impact on its transcription. We have also tested the chromatin density of _BSU1_ in _nrp1-1 nrp2-2_ and found no significant difference compared to wild-type
(Supplementary Fig. 8). To better understand the effects of NRP proteins on transcription, we first focused on the H2A.Z peaks that were dependent on _nrp1-1 nrp2-2_. This analysis showed
that the subset of genes annotated as coding with increased H2A.Z occupancy immediately after the TSS (+1 nucleosome), but not other regions, displayed a slight but significant up-regulation
in _nrp1-1 nrp2-2_ (Supplementary Fig. 9a). This agrees with the fact that lowly expressed genes with high H2A.Z in both the gene body and TSS, on average, show higher expression than lowly
expressed genes with high H2A.Z levels at the gene body but lower H2A.Z levels at the TSS14,35. Next, we examined the global effect of _nrp1-1 nrp2-2_ on transcription. We divided all genes
according to expression levels and noted that, generally, only highly expressed genes were downregulated by _nrp1-1 nrp2-2_ (Supplementary Fig. 9b). This is consistent with previous studies
reporting that highly expressed genes have very low H2.A.Z levels while moderately expressed genes have higher H2A.Z levels14. We then compared the transcriptomes of _arp6-1_ and _nrp1-1
nrp2-2_. Consistent with the phenotype, the overall effect on transcription of _arp6-1_ was larger than that of _nrp1-1 nrp2-2_. When comparing both transcriptomes, we observed that the
number of genes that were downregulated in one but up-regulated in the other (580/317) were marginally higher than the number of genes that were upregulated or downregulated in both
(519/272) (Supplementary Fig. 9c). These data suggest that ARP6 and NRP proteins may perform a counteracting activity in the cell. _NRP1-1 NRP2-2_ DOES NOT AFFECT GLOBAL DISTRIBUTION OF H2A
Since NAP1 was originally described as an H2A-H2B chaperone and NRP proteins have been shown to bind H2A28 (Fig. 2a–c), we wanted to explore if NRP proteins could have any effect on the
deposition of canonical H2A. We previously showed that global levels of H2A were not significantly different between wild-type and _nrp1-1 nrp2-2_ double mutant (Supplementary Fig. 5). To
get more detailed information about H2A abundance and genome-wide distribution, we performed two independent biological replicates of H2A ChIP-Seq. The analysis of metagene plots revealed
that the overall distribution of H2A in Columbia was nearly identical to that of _nrp1-1 nrp2-2_ (Fig. 3b), suggesting that NRP proteins may not play an essential role in the dynamics of
canonical H2A. We did notice an increase of H2A levels in _arp6-1_ and _arp6-1 nrp1-1 nrp2-2_ towards the 5′ end (Fig. 3b). This increase is likely because H2A-H2B replacement by H2A.Z-H2B
in _arp6-1_ backgrounds is severely compromised, meaning that an H2A increase could be expected at regions where H2A.Z is enriched in the wild-type such as the 5′ end of genes. NRP1
CO-LOCALIZES PREDOMINANTLY WITH H2A.Z DEPLETED REGIONS To examine the distribution of the NRP proteins, we performed ChIP experiments with the _9xmyc-NRP1_ complementing line. Although this
approach will assess NRP1 only, we expect that NRP1 and NRP2 co-localize, given the strong interaction between NRP1 and NRP2 in vivo (Table 1 and Supplementary Fig. 4). First, we tested
binding of NRP1-Myc to the putative target loci described previously; _BSU1_, _FLC_. ChIP-qPCR analyses showed a significant enrichment at all these loci compared to the negative control,
(_At1g76840_), and experimental control (No antibody) (Fig. 4a). Then, we examined NRP1 distribution at the genomic level by ChIP-Seq. We found that NRP1 bound mostly, but not exclusively,
to heterochromatic regions that are typically depleted of H2A.Z (Fig. 4b), this agrees with a previous study showing that _YFP-NRP1_ is able to bind to the chromatin of the pericentromeric
loci _Ta3_, _TSI_, and _180_bp repeats28. Consistently, we found that heterochromatic regions displayed the highest increase in H2A.Z occupancy in the _nrp1-1 nrp2-2_ double mutant compared
to wild-type, suggesting an essential role of NRP1 proteins in maintaining low H2A.Z levels around centromeres (Fig. 4b). Next, we examined the occupancy of H2A.Z at the Myc-NRP1 binding
regions in both wild-type and _nrp1-1 nrp2-2_ double mutant plants. When we plotted H2A.Z over highly statistically significant NRP1 peaks in the wild-type (likelihood ratio>1000), we
observed only a minor peak. In contrast, in _nrp1-1 nrp2-2_ double mutants, we observed a substantial H2A.Z peak at the defined NRP1 binding regions (Fig. 4c), suggesting that NRP1 is
localized to areas of heterochromatin and may act to reduce H2A.Z levels at these locations. The overall distribution pattern of both NRP1-Myc and the major gains of H2A.Z in _nrp1-1 nrp2-2_
double mutants resembled that of the histone variant H2A.W, which is primarily centromeric37. In order to explore any possible relationship between NRP proteins and H2A.W, we examined
global levels of H2A.W by western blot in Columbia and _nrp1-1 nrp2-2_ double mutants and did not find any significant changes (Supplementary Fig. 5). Additionally, the H2A.W ChIP signal in
the wild-type did not show any enrichment at _nrp1-1 nrp2-2_ dependent H2A.Z loci (Supplementary Fig. 10). Suggesting that NRP proteins may not play a major role in H2A.W dynamics. NRPS DO
NOT HAVE A CRITICAL EFFECT ON DNA METHYLATION We also analyzed DNA methylation patterns in _nrp1-1 nrp2-2_ mutants. Whole-genome bisulfite-Seq analysis showed that methylation levels are
very similar to those in the wild-type except for a very slight increase in CG and CHG methylation. This excess in methylation appears to occur mainly at transposable elements (TEs)
(Supplementary Fig. 11). Moreover, H2A.Z abundance at TEs appears to be slightly higher in _nrp1-1 nrp2-2_ than in the wild-type (Supplementary Fig. 11e). In summary, the results of this
study identify NRP1 and NRP2 as key factors counteracting the activity of the SWR1 complex. The opposing activities of NRP proteins dependent reduction of H2A.Z and SWR1 deposition of H2A.Z
shape the genomic landscape of H2A.Z and regulate the expression of a subset of genes enriched in H2A.Z near the +1 nucleosome. DISCUSSION Plant species exhibit high levels of plasticity
during development that enables them to adapt and respond in a highly plastic and dynamic fashion to fluctuating environmental conditions, which is especially important due to the sessile
nature of plants. This is presumably the result of genetic factors, but recent evidence suggests that epigenetic changes could play a larger role than previously envisioned. Dynamic changes
in H2A.Z occupancy have been shown to be involved in many aspects of plant biology, many of them in relation to the response to environmental cues5,7,8,35,38. Recently, considerable progress
has been made towards the understanding of H2A.Z removal. In yeast, Inositol Requiring 80 (Ino80) has been shown to be responsible for the exchange of H2A.Z/H2B by H2A/H2B dimers39, and the
Acidic leucine-rich nuclear phosphoprotein 32 family member E (Anp32E) has been shown to remove H2A.Z from the chromatin of mammalian cells40,41,42. However, in plants, the mechanism/s by
which H2A.Z might be removed remains unclear. The role of AtINO80 regarding H2A.Z dynamics has not been clearly established; indeed, it has been shown that AtINO80 is required for H2A.Z
deposition at _FLC_, _MAF4_, and _MAF5_43, while the possible role of ANP32E homolog in plants in regard to H2A.Z is unknown. We have found that _nrp1-1 nrp2-2_ double mutant displays an
early flowering phenotype and affects the expression of genes that are essential for development such as _BSU1_, and the flowering time regulator _FLC_. Interestingly, the expression of
_FLC_ is known to be regulated by the histone variant H2A.Z. Moreover, we have shown that overexpression of _BSU1_ is indeed caused by the disruption of _NRP1_ and _2_ since _BSU1_
expression can be restored to nearly wild-type levels by the addition of either _NRP1_ or _NRP2_-tagged lines. We have also shown functionality by showing that the _nrp1-1 nrp2-2_ double
mutant can rescue the wild-type phenotype of lines overexpressing _BIN2_. These data are in apparent conflict with previous studies performing transcriptomic analyses using _nrp2-1_ instead
of _nrp2-2_ allele28,44. However, these differences in the phenotypes could be explained by differences in the allele strength used in each study. Our findings also showed that the
upregulation of _BSU1_ that we observed in the _nrp1-1 nrp2-2_ double mutant is dependent on the presence of certain levels of H2A.Z as the combination with mutations in genes coding for
three of the core components of SWR1 in Arabidopsis (i.e.,- _ARP6_, _SEF_, and _PIE1_) were able to supress this upregulation. In Arabidopsis three out of twelve genes encoding for H2A and
its variants are classified as H2A.Z according to phylogeny: _HTA8_, _HTA9_, and _HTA11_. Among these three genes _HTA9_ and _HTA11_ show much higher expression levels than _HTA8_; in fact
_hta9-1 hta11-1_ double mutants showed phenotypical and molecular defects typical for plants with reduced levels of H2A.Z6. We found that in the quadruple mutant _hta9-1 hta11-1 nrp1-1
nrp2-2_, _BSU1_ expression showed no substantial difference compared to wild-type, further confirming the requirement of H2A.Z for the _BSU1_ overexpression phenotype of _nrp1-1 nrp2-2_. Our
work confirmed previous reports showing that NRP1 and 2 can bind the canonical H2A28. Indeed, we have confirmed that NRP proteins can be found associated with H2A in vivo. But more
attractively for the focus of this work, our data also showed that NRP proteins strongly interact with H2A.Z in vivo. Although Nap1 in yeast was originally thought to be a histone chaperone
specific for H2A-H2B, it is worth noting that more recent studies in _Schizosaccharomyces pombe_ have shown that tandem affinity purification of the histone variant H2A.Z yielded peptides of
Nap1 and more interestingly the distribution of H2A.Z showed some degree of anticorrelation with that of Nap1, suggesting a role for Nap1 in H2A.Z removal45. We have observed higher levels
of H2A.Z in _nrp1-1 nrp2-2_ compared to wild-type. This excess of H2A.Z was found to some extent in chromosome arms and gene coding regions, but it mainly localized around heterochromatic
regions and centromeres, where H2A.Z is usually absent. Interestingly, overall gains of H2A.Z in _nrp1-1 nrp2-2_ double mutants generally co-localized with NRP1. The fact that both H2A.Z
gains in _nrp1-1 nrp2-2_ and NRP1 binding sites are largely concentrated in heterochromatic regions suggests that the primary function of NRP proteins might be the regulation of H2A.Z levels
at these particular regions. Alternatively, it is possible that NRP proteins also play a role in euchromatin but higher SWR1 activity and/or nucleosome turnover in the chromosome arms
caused by transcription, recombination, etc… may mask the activity of NRP proteins or make more difficult the detection of NRP1 and H2A.Z gains by ChIP. In any case, these data, together
with the genetic and biochemical data presented, suggests a connection between NRP proteins and reduced levels of H2A.Z. There are at least three possible explanations for the increase in
H2A.Z in _nrp1-1 nrp2-2_. One possibility is that NRP proteins simply evict H2A.Z-containing nucleosomes at discrete loci in the genome and mainly near the centromeres. A second possible
scenario would be that NRP proteins would not evict H2A.Z-containing nucleosomes but instead replace H2A.Z with H2A. A third alternative could be that NRP proteins deposit H2A, H2A.W, or
H2A.X, and in their absence, H2A.Z would be deposited instead by SWR1 complex, (or an unknown H2A.Z chaperone). Although further work is needed to elucidate the precise mechanism of NRP
proteins, our results somewhat favor the first hypothesis for a few reasons. The H2A.Z to H2A exchange model would involve lower levels of H2A at regions of the genome in which we observed
excessive levels of H2A.Z in _nrp1-1 nrp2-2_, instead, we did not observe any significant effect on the distribution of H2A in _nrp1-1 nrp2-2_. This would also be an argument against NRP
proteins being responsible for H2A deposition. The available evidence also does not support a role for NRPs in H2A.X deposition. H2A.X is thought to be mainly involved in meiosis, and its
rapid accumulation is known to be an early mark of homologous recombination (HR) foci formation. The lack of NRP proteins impairs HR and the response to double-strand breaks (DSB)30,46, in
fact, regarding HR, NRP proteins are epistatic over AtINO80, which seems to act downstream46. Also, NRP1 quickly accumulates after the treatment with the DSB-inducing drug CPT30, which
suggests a role for NRP proteins in HR. However, upon loss of NRP proteins, no substantial effect is observed in the deposition of H2A.X during DNA repair at HR foci. Interestingly, in yeast
and mammalian cells, the removal of H2A.Z is an essential step for proper HR during DNA repair after DSB47,48. Thus, it is likely that the HR phenotype observed in the _nrp1-1 nrp2-1_ might
be due to the inability to remove H2A.Z rather than a defect in H2A.X deposition, and it is possible that NRP proteins and AtINO80 cooperate in the removal of H2A.Z immediately after DSBs.
Although it is still unknown which protein/protein complex deposits H2A.W in Arabidopsis, it is known that H2A.W is virtually absent from coding genes37 while we do find NRP1 outside of the
centromeres. Moreover, global levels of H2A.W appear to be unaffected in _nrp1-1 nrp2-2_ double mutants. METHODS PLANT MATERIALS All the lines used in this article are in Columbia-0
background. Plants were grown under standard long day conditions unless stated differently. The mutant line _nrp2-2_ is Salk_205276. MRNA SEQUENCING Total RNA was purified from 100 mg of
whole plant tissue (14DAG), then ground and extracted using Trizol (Invitrogen, USA). Trizol supernatant was further purified by RNeasy columns (Qiagen, Germany). The mRNA was isolated by
magnetic Oligo (dT) beads, fragmentation buffer was then added. 6 bp random hexamers were used to synthesize single-stranded cDNA, double-strand cDNA then was synthesized by DNA Pol I.
AMPure XP beads (Beckman Coulter, USA) were used to purify ds cDNA. 3′ Adenine and sequencing adaptors were added. AMPure XP beads were used to select the size of each segment. Finally, PCR
was used to enrich the segments to obtain the cDNA library. mRNA library sequencing was done by NovoGene (Beijing, China). REAL-TIME QUANTITATIVE PCR We prepared Total RNA from whole
seedlings tissue (14DAG) using RNA Prep Pure Plant Kit (Tiangen, China). We used ~2 mg of RNA for the synthesis of cDNA using PrimeScript Reagent Kit with gDNA Eraser (Takara, Japan). We
performed signal detection, quantification, and normalization using Quant Studio 6 proprietary Software (Life technologies, USA). For all the quantitative analyses, we performed a minimum of
three biological replicates, three technical replicates each. CO-IMMUNOPRECIPITATION ASSAYS Anti-H2A or anti-H2A.Z polyclonal antibodies were raised in rabbits using the following peptides:
SGKGAKGLIMGKPSGSDKDKDKKKPIT-C/AGKGGKGLVAAKTMAANKDKDKDKKKPIS-C for H2A.Z and the peptides C-RGKTLGSGSAKKATTR and C-RGKTLGSGVAKKSTSR for H2A as an antigen (AbClonal, China). We coupled the
antibody to magnetic beads using the Dynabeads antibody coupling kit (Invitrogen, USA). We ground ~2 g of 14 days old seedlings in liquid nitrogen. The we added 10 ml of IP buffer (50 mM
Tris pH 7.6, 150 mM NaCl, 5 mM MgCl2, 10% glycerol, 0.1% NP-40, 0.5 mM DTT, 1 mM β-mercaptoethanol, 1 mM PMSF, and 1xPlant Protease Inhibitors cocktail (SIGMA, USA)). We incubated the mix on
ice for 20 min, then spun at 4000 xg for 10 min at 4 °C. After that, we passed the supernatant through two layers of Miracloth (Millipore, USA). Then, we added Anti-H2A or H2A.Z antibody
coupled to magnetic beads. We incubated the mix overnight at 4 °C with gentle rotation. The next morning, we washed the beads six times with IP buffer, 5 min per wash at 4 °C with gentle
rotation, and then incubated at 100 °C for 15 min. We performed western blots and detection using a dilution of 1: 1000 anti-Myc 9E10 mouse monoclonal antibody (Santa Cruz Biotechnology,
USA), or a dilution of 1: 1000 of anti-Flag-HRP antibody (Sigma, USA). In the case of NRP2-3xFlag and 9xMyc-NRP1 co-IP, we used anti c-Myc magnetic beads (Pierce, USA). The following steps
were identical to H2A or H2A.Z co-IP. PROTEIN EXPRESSION ANALYSIS Specific antibodies against proteins were used to study their expression. In this study we have used the following
antibodies: Anti-Ub SC8017 (Santa Cruz Biotechnology, USA), anti-GFP N598 (MBL, Japan), anti-H3 ab1791 (Abcam, USA), anti-H2A, anti-H2A.Z, (please see Co-immunoprecipitation assays section),
and anti-H2A.W49. We analyzed the expression of proteins using western hybridizations. For total protein extracts, we ground about 100 mg of fresh tissue. We then transferred the powder to
a tube containing 250μL of protein extraction buffer (50 mM Tris HCl, pH-7.4, 1%β-mercaptoethanol, 12% sucrose, 0.1% Triton X-100, 5 mM PMSF). We stirred and then centrifuged the mixture for
5 min at 12,000 x _g_ at 4 °C. We transferred the supernatant containing the proteins to a fresh tube. We repeated this step twice. Before loading, we mixed the samples with an equal volume
of 2x Laemmli loading buffer (100 mM Tris-HCl, pH = 6.8, 200 mM DTT, 4% SDS, 20% glycerol, 0.1% bromophenol blue) and then denatured the proteins by boiling the mixture for 15 min. For
histone extraction we used the EpiQuikTM total histone extraction kit (EPIGENTEK, USA) and followed manufacturer recommendations. We loaded between 10-20μg of total proteins into 4–12% in
the case of total protein or 12% acrylamide gels in the case of histone extracts (Invitrogen, USA) and transferred to an Immobilon-P membrane (Millipore, USA) following the instructions from
the manufacturer. The antibody dilution used in each case is as follows: Anti-Ub (1:200), anti-GFP (1:2000), anti-H3 (1:5000), anti-H2A (1:1000), anti-H2A.Z (1:1000), and for anti-H2A.W we
followed the dilution suggested by the authors49. We detected proteins using the Novex™ ECL Chemiluminescent Substrate Reagent kit (Invitrogen, USA) following the manufacturer
recommendations. IMMUNOPURIFICATION FOR MASS SPECTROMETRY We collected between 5 and 8.5 g tissue from young inflorescences from the complementing lines T3 _NRP2-3xFlag_ or T3 _NRP2-9xMyc_
and from Columbia plants as negative controls. Tissue was ground to a fine powder using a RETCH tissue-lyser and suspended in 20–35 mL of IP buffer, then centrifuged for 5 min at 4000 x _g_
and 4 °C. The lysate was filtered through two layers of Miracloth. For _NRP2-3xFlag_, the supernatant was incubated with 250 μL FLAG M2 magnetic beads (Sigma, USA), at 4 °C for 2 h. The
beads were then washed initially with 10 mL of IP buffer, then five additional times (5 min rotating at 4 °C with 1 mL IP buffer). The FLAG-IP was eluted twice with 400 µl of 250 µgmL−1 3X
FLAG peptides (Sigma, USA) in 1xPBS pH = 7.4 (2.7 mM KCl, 150 mM NaCl, 10 mM Na2HPO4, 1.8 mM K2HPO4), rotating for 15 min. at 4 °C. The eluted protein complexes were precipitated by the
addition of 25% TCA, washed twice in ice-cold acetone and subjected to mass spectrometric analyses. For _NRP2-9xMyc_ supernatant was incubated with 500 μL streptavidin-coupled magnetic beads
for 1 h at 4 °C (Invitrogen, USA). Proteins were released from the streptavidin beads by 3C cleavage overnight at 4 °C. Then 3C-GST (SIGMA, USA) was removed by incubation with 300 μL of
GST-coupled magnetic beads (Pierce, USA) twice rotating for 30 min. at 4 °C. The following steps were identical to _NRP2-3xFlag_. TCA precipitates were digested by the sequential addition of
lys-C and trypsin proteases50. The digested peptides were fractionated online using reversed-phase chromatography and eluted directly into a Fusion Lumos mass spectrometer (Thermofisher,
USA) where MS/MS spectra were collected51. Database searching was performed with the ProLuCID algorithm against a fasta protein database downloaded from The Arabidopsis Information
Resource52. Peptide identification and protein mapping were performed using the DTASelect2 algorithm53. Peptide spectrum matches were filtered using a false-positive rate of less than 5% as
estimated by a decoy database strategy and confident protein identifications required at least two unique peptides per protein54. CHROMATIN IMMUNOPRECIPITATION ASSAYS For canonical H2A,
H2A.Z, and Myc ChIP-Seq, we collected 2 g, 2 g, and 5 g, respectively of 14 days old seedlings grown on 1x MS plates plus 1% sucrose under long-day conditions. Plants were crosslinked in 1%
Formaldehyde solution under intermittent vacuum until they became translucent, crosslinking was stopped with glycine to a final concentration of 125 mM, and then seedlings were washed in
milli-Q water, blotted using Kim wipes and ground to a fine powder in a tissue lyser. The powder was then suspended in Honda Buffer (0.45 M Sucrose, 1.25% Ficoll, 2.5% Dextran, 20 mM HEPES
pH7.4, 10 mM MgCl2, 0.5% TritonX-100, 5 mM DTT, 1 m MPMSF, and 1xPlant protease inhibitor cocktail (SIGMA, USA), incubated on ice for 20 min and filtered through two layers of Miracloth
(Millipore, USA). The mix was centrifuged for 15 min at 2000 x _g_ at 4 °C, the supernatant was discarded, and the pellet was again carefully resuspended into Honda Buffer. This step was
repeated until pellet appeared completely white. The white pellet containing nuclei was then resuspended in Nuclei Lysis Buffer (50 mM TrisHCl pH = 8, 10 mM EDTA, 1% SDS, 1 mM PMSF, and
1xPlant protease inhibitor cocktail (SIGMA, USA), incubated on ice for 20 min. and sheared using Bioruptor Plus (Diagenode, USA) 30 s ON/OFF, Max power, 21 cycles at 4 °C. Chromatin was
diluted tenfold in ChIP Dilution Buffer (167 mM NaCl, 16.7 mM TrisHCl pH8 1.2 mM EDTA, and 1.1% Triton X-100). Antibody coupled to Dynabeads was added to diluted chromatin and incubated
overnight at 4 °C with gentle rotation. After that beads were recovered and washed 6 times with Washing Buffer (150 mM NaCl, 20 mM TrisHCl pH8 2 mM EDTA, 1% Triton X-100, 0,1% SDS, and 1 mM
PMSF). Washed beads were then boiled for 10 min. in the presence of 10% Chelex (BioRad, USA) to reverse crosslink, then digested using 20 µg of proteinase K for an hour at 43 °C. After
digestion, DNA was recovered using MinElute Spin Columns (Qiagen, Germany) following manufacturer instructions. Libraries were generated with NuGEN Ovation Ultra Low System V2 kit, according
to the manufacturer’s instructions, and were sequenced on an Illumina HiSeq 4000 instrument. In the case of Myc ChIP-qPCR everything was done in the same way except that after incubation
anti-Myc antibody was pulled down using Protein A/G magnetic beads (Pierce, USA). For ChIP-qPCR at least three biological replicates were performed, three technical replicates each. MRNA AND
CHIP-SEQ BIOINFORMATIC ANALYSES ChIP-Seq reads were aligned to the reference genome (TAIR10) with Bowtie (v1.1.2), allowing only uniquely mapping reads with 0 mismatches. Duplicated reads
were removed by Samtools55. ChIP-Seq peaks were called by MACS256 (v2.1.1.) and annotated with ChIPseeker57. Differential peaks with likelihood ratio > 1000 were called by bdgdiff
function in MACS2. Metaplots of ChIP-Seq data were plotted by deeptools (v2.5.1). To evaluate the influence of H2A.Z differential peaks to gene expression, we annotated differential peaks
with ChIPseeker, and compared expression level of differential peaks’ proximal genes between Col-0 and mutants. WHOLE-GENOME BISULFITE SEQUENCING AND ANALYSIS Total DNA was extracted from
plant (leaf) tissue (14DAG) with DNeasy kit (Qiagen, USA). Genomic DNA was converted with bisulfite treatment with EpiTect Bisulfite Kit (Qiagen, USA). Whole-genome Bisulfite Sequencing
library sequencing was done by NovoGene (Beijing, China). BS-seq reads were mapped to TAIR10 reference genome by bsmap (v2.90) with allowing 2 mismatches and 1 best hit (-v 2 -w 1). Reads
with three or more consecutive methylated CHH sites were considered as “unconverted” and subsequently removed. DNA methylation levels were calculated by #C/ (#C + #T). Whole-genome
methylation was plotted with ViewBS (v0.1.8). REPORTING SUMMARY Further information on research design is available in the Nature Research Reporting Summary linked to this article. DATA
AVAILABILITY Data supporting the findings of this work are available within the paper and its Supplementary Information files. A reporting summary for this article is available as a
Supplementary Information file. The datasets generated and analyzed during the current study are available from the corresponding author upon request. All high-throughput sequencing data
generated in this study are available at NCBI’s Gene Expression Omnibus under accession number GSE127986 [https://www.ncbi.nlm.nih.gov/geo/query/acc.cgi?acc=GSE127986]. The mass spectrometry
proteomics data have been deposited to the ProteomeXchange Consortium via the PRIDE58 partner repository with the dataset identifier PXD019248
[https://www.ebi.ac.uk/pride/archive/projects/PXD019248]. The source data underlying Figs. 1b–d, 2, 3a, e, f, 4a, b, Table 1, as well as Supplementary Figs. 1–6, 8, 9, and 11 are provided as
a Source Data file. Source data are provided with this paper. REFERENCES * Cutter, A. R. & Hayes, J. J. A brief review of nucleosome structure. _FEBS Lett._ 589, 2914–2922 (2015).
Article CAS PubMed PubMed Central Google Scholar * Talbert, P. B. & Henikoff, S. Histone variants-ancient wrap artists of the epigenome. _Nat. Rev. Mol. Cell Biol._ 11, 264–275
(2010). Article CAS PubMed Google Scholar * Berriri, S., Gangappa, S. N. & Kumar, S. V. SWR1 Chromatin-remodeling complex subunits and H2A.Z have non-overlapping functions in
immunity and gene regulation in Arabidopsis. _Mol. Plant_ 9, 1051–1065 (2016). Article CAS PubMed Google Scholar * Choi, K. et al. Arabidopsis meiotic crossover hot spots overlap with
H2A.Z nucleosomes at gene promoters. _Nat. Genet_ 45, 1327–1336 (2013). Article CAS PubMed Google Scholar * Kumar, S. V. & Wigge, P. A. H2A.Z-containing nucleosomes mediate the
thermosensory response in Arabidopsis. _Cell_ 140, 136–147 (2010). Article CAS PubMed Google Scholar * March-Diaz, R. et al. Histone H2A.Z and homologues of components of the SWR1
complex are required to control immunity in Arabidopsis. _Plant J._ 53, 475–487 (2008). Article CAS PubMed Google Scholar * Smith, A. P. et al. Histone H2A.Z regulates the expression of
several classes of phosphate starvation response genes but not as a transcriptional activator. _Plant Physiol._ 152, 217–225 (2010). Article CAS PubMed PubMed Central Google Scholar *
Sura, W. et al. Dual role of the histone variant H2A.Z in transcriptional regulation of stress-response Genes. _Plant Cell_ 29, 791–807 (2017). Article CAS PubMed PubMed Central Google
Scholar * Talbert, P. B. & Henikoff, S. Environmental responses mediated by histone variants. _Trends Cell Biol._ 24, 642–650 (2014). Article CAS PubMed Google Scholar * Zhao, L. et
al. KLU suppresses megasporocyte cell fate through SWR1-mediated activation of WRKY28 expression in Arabidopsis. _Proc. Natl Acad. Sci. USA_ 115, E526–E535 (2018). Article CAS PubMed
Google Scholar * Zlatanova, J. & Thakar, A. H2A.Z: view from the top. _Structure_ 16, 166–179 (2008). Article CAS PubMed Google Scholar * Deal, R. B., Topp, C. N., McKinney, E. C.
& Meagher, R. B. Repression of flowering in Arabidopsis requires activation of FLOWERING LOCUS C expression by the histone variant H2A.Z. _Plant Cell_ 19, 74–83 (2007). Article CAS
PubMed PubMed Central Google Scholar * Zilberman, D., Coleman-Derr, D., Ballinger, T. & Henikoff, S. Histone H2A.Z and DNA methylation are mutually antagonistic chromatin marks.
_Nature_ 456, 125–129 (2008). Article ADS CAS PubMed PubMed Central Google Scholar * Gomez-Zambrano, A., Merini, W. & Calonje, M. The repressive role of Arabidopsis H2A.Z in
transcriptional regulation depends on AtBMI1 activity. _Nat. Commun._ 10, 2828 (2019). Article ADS PubMed PubMed Central CAS Google Scholar * Kobor, M. S. et al. A protein complex
containing the conserved Swi2/Snf2-related ATPase Swr1p deposits histone variant H2A.Z into euchromatin. _PLoS Biol._ 2, E131 (2004). Article PubMed PubMed Central Google Scholar *
Mizuguchi, G. et al. ATP-driven exchange of histone H2AZ variant catalyzed by SWR1 chromatin remodeling complex. _Science_ 303, 343–348 (2004). Article ADS CAS PubMed Google Scholar *
Altaf, M. et al. NuA4-dependent acetylation of nucleosomal histones H4 and H2A directly stimulates incorporation of H2A.Z by the SWR1 complex. _J. Biol. Chem._ 285, 15966–15977 (2010).
Article CAS PubMed PubMed Central Google Scholar * Ranjan, A. et al. Nucleosome-free region dominates histone acetylation in targeting SWR1 to promoters for H2A.Z replacement. _Cell_
154, 1232–1245 (2013). Article CAS PubMed Google Scholar * Yen, K., Vinayachandran, V. & Pugh, B. F. SWR-C and INO80 chromatin remodelers recognize nucleosome-free regions near +1
nucleosomes. _Cell_ 154, 1246–1256 (2013). Article CAS PubMed PubMed Central Google Scholar * Potok, M. E. et al. Arabidopsis SWR1-associated protein methyl-CpG-binding domain 9 is
required for histone H2A.Z deposition. _Nat. Commun._ 10, 3352 (2019). Article ADS CAS PubMed PubMed Central Google Scholar * Sijacic, P., Holder, D. H., Bajic, M. & Deal, R. B.
Methyl-CpG-binding domain 9 (MBD9) is required for H2A.Z incorporation into chromatin at a subset of H2A.Z-enriched regions in the Arabidopsis genome. _PLoS Genet_ 15, e1008326 (2019).
Article CAS PubMed PubMed Central Google Scholar * Laskey, R. A., Honda, B. M., Mills, A. D. & Finch, J. T. Nucleosomes are assembled by an acidic protein which binds histones and
transfers them to DNA. _Nature_ 275, 416–420 (1978). Article ADS CAS PubMed Google Scholar * Levchenko, V. & Jackson, V. Histone release during transcription: NAP1 forms a complex
with H2A and H2B and facilitates a topologically dependent release of H3 and H4 from the nucleosome. _Biochemistry_ 43, 2359–2372 (2004). Article CAS PubMed Google Scholar * Lorch, Y.,
Maier-Davis, B. & Kornberg, R. D. Chromatin remodeling by nucleosome disassembly _in vitro_. _Proc. Natl Acad. Sci. USA_ 103, 3090–3093 (2006). Article ADS CAS PubMed PubMed Central
Google Scholar * Gao, J. et al. NAP1 family histone chaperones are required for somatic homologous recombination in Arabidopsis. _Plant Cell_ 24, 1437–1447 (2012). Article CAS PubMed
PubMed Central Google Scholar * Dong, A. et al. Regulation of biosynthesis and intracellular localization of rice and tobacco homologues of nucleosome assembly protein 1. _Planta_ 216,
561–570 (2003). Article CAS PubMed Google Scholar * Bíró, J. D. M. & Fehér, A. NAP-Related Protein 1 (Atnrp1) overexpression increases the heat tolerance of Arabidopsis
cells/plantlets. _J. Plant Biochem. Physiol._ 1, 115 (2013). Google Scholar * Zhu, Y. et al. Arabidopsis NRP1 and NRP2 encode histone chaperones and are required for maintaining
postembryonic root growth. _Plant Cell_ 18, 2879–2892 (2006). Article CAS PubMed PubMed Central Google Scholar * Liu, Z. et al. Molecular and reverse genetic characterization of
NUCLEOSOME ASSEMBLY PROTEIN1 (NAP1) genes unravels their function in transcription and nucleotide excision repair in _Arabidopsis thaliana_. _Plant J._ 59, 27–38 (2009). Article CAS PubMed
Google Scholar * Gonzalez-Arzola, K. et al. Histone chaperone activity of _Arabidopsis thaliana_ NRP1 is blocked by cytochrome c. _Nucleic Acids Res_. 45, 2150–2165 (2017). Article CAS
PubMed Google Scholar * Mora-Garcia, S. et al. Nuclear protein phosphatases with kelch-repeat domains modulate the response to brassinosteroids in Arabidopsis. _Gene Dev._ 18, 448–460
(2004). Article CAS PubMed PubMed Central Google Scholar * Kim, T. W., Guan, S. H., Burlingame, A. L. & Wang, Z. Y. The CDG1 kinase mediates brassinosteroid signal transduction from
BRI1 receptor kinase to BSU1 phosphatase and GSK3-like kinase BIN2. _Mol. Cell_ 43, 561–571 (2011). Article CAS PubMed PubMed Central Google Scholar * Karlova, R. et al. The
Arabidopsis SOMATIC EMBRYOGENESIS RECEPTOR-LIKE KINASE1 protein complex includes BRASSINOSTEROID-INSENSITIVE1. _Plant Cell_ 18, 626–638 (2006). Article CAS PubMed PubMed Central Google
Scholar * Kumar, A., Kumar Singh, A., Chandrakant Bobde, R. & Vasudevan, D. Structural characterization of Arabidopsis thaliana NAP1-RELATED PROTEIN 2 (AtNRP2) and comparison with its
homolog AtNRP1. _Molecules_ 24, 2258 (2019). Article CAS PubMed Central Google Scholar * Coleman-Derr, D. & Zilberman, D. Deposition of histone variant H2A.Z within gene bodies
regulates responsive genes. _PLoS Genet_ 8, e1002988 (2012). Article CAS PubMed PubMed Central Google Scholar * Choi, K. et al. Arabidopsis homologs of components of the SWR1 complex
regulate flowering and plant development. _Development_ 134, 1931–1941 (2007). Article CAS PubMed Google Scholar * Yelagandula, R. et al. The histone variant H2A.W defines
heterochromatin and promotes chromatin condensation in Arabidopsis. _Cell_ 158, 98–109 (2014). Article CAS PubMed PubMed Central Google Scholar * Zahraeifard, S. et al. Rice H2A.Z
negatively regulates genes responsive to nutrient starvation but promotes expression of key housekeeping genes. _J. Exp. Bot._ 69, 4907–4919 (2018). Article CAS PubMed PubMed Central
Google Scholar * Papamichos-Chronakis, M., Watanabe, S., Rando, O. J. & Peterson, C. L. Global regulation of H2A.Z localization by the INO80 chromatin-remodeling enzyme is essential for
genome integrity. _Cell_ 144, 200–213 (2011). Article CAS PubMed PubMed Central Google Scholar * Gursoy-Yuzugullu, O., Ayrapetov, M. K. & Price, B. D. Histone chaperone Anp32e
removes H2A.Z from DNA double-strand breaks and promotes nucleosome reorganization and DNA repair. _Proc. Natl Acad. Sci. USA_ 112, 7507–7512 (2015). Article ADS CAS PubMed PubMed
Central Google Scholar * Mao, Z. et al. Anp32e, a higher eukaryotic histone chaperone directs preferential recognition for H2A.Z. _Cell Res_. 24, 389–399 (2014). Article CAS PubMed
PubMed Central Google Scholar * Obri, A. et al. ANP32E is a histone chaperone that removes H2A.Z from chromatin. _Nature_ 505, 648–653 (2014). Article ADS CAS PubMed Google Scholar *
Zhang, C. et al. The chromatin-remodeling factor AtINO80 plays crucial roles in genome stability maintenance and in plant development. _Plant J._ 82, 655–668 (2015). Article CAS PubMed
Google Scholar * Barna, B. et al. Arabidopsis NAP-related proteins (NRPs) contribute to the coordination of plant growth, developmental rate, and age-related pathogen resistance under short
days. _Plant Sci._ 267, 124–134 (2018). Article CAS PubMed Google Scholar * Buchanan, L. et al. The Schizosaccharomyces pombe JmjC-protein, Msc1, prevents H2A.Z localization in
centromeric and subtelomeric chromatin domains. _PLoS Genet_ 5, e1000726 (2009). Article PubMed PubMed Central CAS Google Scholar * Zhou, W. et al. Distinct roles of the histone
chaperones NAP1 and NRP and the chromatin-remodeling factor INO80 in somatic homologous recombination in Arabidopsis thaliana. _Plant J._ 88, 397–410 (2016). Article CAS PubMed Google
Scholar * Alatwi, H. E. & Downs, J. A. Removal of H2A.Z by INO80 promotes homologous recombination. _EMBO Rep._ 16, 986–994 (2015). Article CAS PubMed PubMed Central Google Scholar
* Lademann, C. A., Renkawitz, J., Pfander, B. & Jentsch, S. The INO80 complex removes H2A.Z to promote presynaptic filament formation during homologous recombination. _Cell Rep._ 19,
1294–1303 (2017). Article CAS PubMed Google Scholar * Osakabe, A. et al. Histone H2A variants confer specific properties to nucleosomes and impact on chromatin accessibility. _Nucleic
Acids Res_. 46, 7675–7685 (2018). Article CAS PubMed PubMed Central Google Scholar * Heinzelman, P., Powers, D. N., Wohlschlegel, J. A. & John, V. Shotgun proteomic profiling of
bloodborne nanoscale extracellular vesicles. _Methods Mol. Biol._ 1897, 403–416 (2019). Article CAS PubMed Google Scholar * Levy, M. J., Washburn, M. P. & Florens, L. Probing the
Sensitivity of the orbitrap lumos mass spectrometer using a standard reference protein in a complex background. _J. Proteome Res_. 17, 3586–3592 (2018). Article CAS PubMed PubMed Central
Google Scholar * Xu, T. et al. ProLuCID: An improved SEQUEST-like algorithm with enhanced sensitivity and specificity. _J. Proteom._ 129, 16–24 (2015). Article CAS Google Scholar *
Cociorva, D., Tabb, D. L. & Yates, J. R. Validation of tandem mass spectrometry database search results using DTASelect. _Curr. Protoc. Bioinforma._ 13, 14 (2007). Google Scholar *
Elias, J. E. & Gygi, S. P. Target-decoy search strategy for increased confidence in large-scale protein identifications by mass spectrometry. _Nat. Methods_ 4, 207–214 (2007). Article
CAS PubMed Google Scholar * Li, H. et al. The Sequence Alignment/Map format and SAMtools. _Bioinformatics_ 25, 2078–2079 (2009). Article PubMed PubMed Central CAS Google Scholar *
Zhang, Y. et al. Model-based analysis of ChIP-Seq (MACS). _Genome Biol_. 9, R137 (2008). Article PubMed PubMed Central CAS Google Scholar * Yu, G., Wang, L. G. & He, Q. Y.
ChIPseeker: an R/Bioconductor package for ChIP peak annotation, comparison and visualization. _Bioinformatics_ 31, 2382–2383 (2015). Article CAS PubMed Google Scholar * Perez-Riverol, Y.
et al. The PRIDE database and related tools and resources in 2019: improving support for quantification data. _Nucleic Acids Res_. 47, D442–D450 (2019). Article CAS PubMed Google Scholar
Download references ACKNOWLEDGEMENTS We want to thank Wenfei Wang and Jianming Li for the donation of the lines _bri-116_ and _BIN2::GFP_, respectively. H2A.W antibodies were a kind gift
from Frederic Berger. We are also grateful to Yasaman Jami-Alahmadi and Na Hui for technical support. The authors are indebted to Xiaofeng Cao as well. The work in the I.A. laboratory was
supported by the National Science Foundation of China (grant numbers: 31571328, 31801025 and 31870270), and the work in the S.E.J. laboratory was supported by NIH grant R35 GM130272. S.E.J.
is an investigator of the Howard Hughes Medical Institute. AUTHOR INFORMATION Author notes * These authors contributed equally: Yafei Wang, Zhenhui Zhong. AUTHORS AND AFFILIATIONS * State
Key Laboratory of Crop Stress Biology for Arid Areas and College of Life Sciences, Northwest A&F University, Yangling, 712100, Shaanxi, China Yafei Wang & Israel Ausin * Department
of Molecular, Cell and Developmental Biology, University of California, Los Angeles, CA, 90095, USA Zhenhui Zhong, Suhua Feng & Steven E. Jacobsen * State Key Laboratory of Ecological
Pest Control for Fujian and Taiwan Crops, College of Plant Protection, Fujian Agriculture and Forestry University, Fuzhou, 350002, China Zhenhui Zhong & Zonghua Wang * Haixia Institute
of Science and Technology, Fujian Agriculture and Forestry University, Fuzhou, 350002, China Yaxin Zhang * Leibniz Institute of Plant Genetics and Crop Plant Research (IPK), OT Gatersleben,
Stadt Seeland, 06466, Germany Linhao Xu * Department of Biological Chemistry, David Geffen School of Medicine, University of California, Los Angeles, CA, 90095, USA Shima Rayatpisheh &
James A. Wohlschlegel * Howard Hughes Medical Institute, University of California, Los Angeles, CA, 90095, USA Steven E. Jacobsen Authors * Yafei Wang View author publications You can also
search for this author inPubMed Google Scholar * Zhenhui Zhong View author publications You can also search for this author inPubMed Google Scholar * Yaxin Zhang View author publications You
can also search for this author inPubMed Google Scholar * Linhao Xu View author publications You can also search for this author inPubMed Google Scholar * Suhua Feng View author
publications You can also search for this author inPubMed Google Scholar * Shima Rayatpisheh View author publications You can also search for this author inPubMed Google Scholar * James A.
Wohlschlegel View author publications You can also search for this author inPubMed Google Scholar * Zonghua Wang View author publications You can also search for this author inPubMed Google
Scholar * Steven E. Jacobsen View author publications You can also search for this author inPubMed Google Scholar * Israel Ausin View author publications You can also search for this author
inPubMed Google Scholar CONTRIBUTIONS Y.W. Performed ChIP and Co-IP and generated NRP1-tagged transgenic lines. Y.W. and L.X. performed genetic and expression analysis. Y.Z. generated
NRP2-tagged transgenic lines and performed IP. L.X., Z.Z., and S.F. generated WGBS libraries. Z.Z. and S.F. generated ChIP-Seq sequencing libraries and performed high-throughput sequencing.
Z.Z. performed bioinformatics analyses of sequencing data. S.R. and J.A.W. performed mass spectrometry. S.E.J. and I.A. coordinated the research. Z.W, S.E.J. and I.A. wrote the manuscript,
with contributions from all other authors. CORRESPONDING AUTHORS Correspondence to Steven E. Jacobsen or Israel Ausin. ETHICS DECLARATIONS COMPETING INTERESTS The authors declare no
competing interests. ADDITIONAL INFORMATION PEER REVIEW INFORMATION _Nature Communications_ thanks the anonymous reviewers for their contribution to the peer review of this work. Peer
reviewer reports are available. PUBLISHER’S NOTE Springer Nature remains neutral with regard to jurisdictional claims in published maps and institutional affiliations. SUPPLEMENTARY
INFORMATION SUPPLEMENTARY INFORMATION PEER REVIEW FILE REPORTING SUMMARY SOURCE DATA SOURCE DATA RIGHTS AND PERMISSIONS OPEN ACCESS This article is licensed under a Creative Commons
Attribution 4.0 International License, which permits use, sharing, adaptation, distribution and reproduction in any medium or format, as long as you give appropriate credit to the original
author(s) and the source, provide a link to the Creative Commons license, and indicate if changes were made. The images or other third party material in this article are included in the
article’s Creative Commons license, unless indicated otherwise in a credit line to the material. If material is not included in the article’s Creative Commons license and your intended use
is not permitted by statutory regulation or exceeds the permitted use, you will need to obtain permission directly from the copyright holder. To view a copy of this license, visit
http://creativecommons.org/licenses/by/4.0/. Reprints and permissions ABOUT THIS ARTICLE CITE THIS ARTICLE Wang, Y., Zhong, Z., Zhang, Y. _et al._ NAP1-RELATED PROTEIN1 and 2 negatively
regulate H2A.Z abundance in chromatin in Arabidopsis. _Nat Commun_ 11, 2887 (2020). https://doi.org/10.1038/s41467-020-16691-x Download citation * Received: 18 October 2019 * Accepted: 20
May 2020 * Published: 08 June 2020 * DOI: https://doi.org/10.1038/s41467-020-16691-x SHARE THIS ARTICLE Anyone you share the following link with will be able to read this content: Get
shareable link Sorry, a shareable link is not currently available for this article. Copy to clipboard Provided by the Springer Nature SharedIt content-sharing initiative