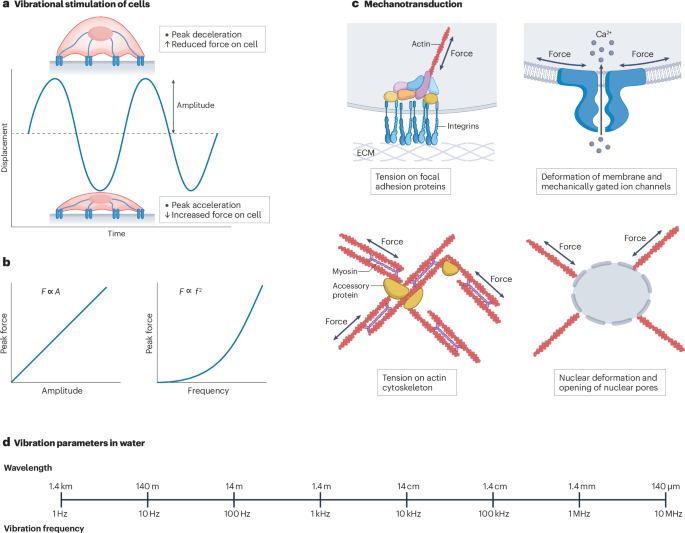
- Select a language for the TTS:
- UK English Female
- UK English Male
- US English Female
- US English Male
- Australian Female
- Australian Male
- Language selected: (auto detect) - EN
Play all audios:
ABSTRACT Cell engineering has the aim of producing cells with controlled phenotype for medical use, for example, for cell therapy, cell transplantation or drug discovery. However, chemical
induction of cell phenotypes, in particular, the use of inductive media and growth factors, often lacks specificity, might be unsuitable for clinical use and remains costly and difficult to
scale up. Alternatively, mechanotransductive stimulation can be applied to engineer cells with specific phenotypes. In this Review, we discuss vibration as a mechanotransductive
cell-engineering tool for both in vitro phenotypic control and in vivo regenerative therapy. We examine how vibration devices can be designed to provide specific frequencies and amplitudes
to which cells respond through either adhesion-induced or channel-induced mechanotransduction pathways. We further highlight key applications of vibrational stimulation for bone regeneration
as well as whole-body vibration as regenerative therapy, identifying important mechanisms of action and gaps in translational pipelines. KEY POINTS * Cells can respond to their mechanical
environment through phenotypic and functional changes. * Vibration is being explored for cell engineering to modify cellular growth, motility and differentiation. * Various vibration
parameters (frequency, amplitude and duration) are being explored for cell engineering, but their relation to mechanotransductive signalling and phenotypic changes are yet to be fully
understood. * Vibrational stimulation might be clinically applied for cell therapy or regenerative medicine, but scalibility and optimization of parameters remain challenging. Access through
your institution Buy or subscribe This is a preview of subscription content, access via your institution ACCESS OPTIONS Access through your institution Subscribe to this journal Receive 12
digital issues and online access to articles $119.00 per year only $9.92 per issue Learn more Buy this article * Purchase on SpringerLink * Instant access to full article PDF Buy now Prices
may be subject to local taxes which are calculated during checkout ADDITIONAL ACCESS OPTIONS: * Log in * Learn about institutional subscriptions * Read our FAQs * Contact customer support
SIMILAR CONTENT BEING VIEWED BY OTHERS ULTRASOUND-ASSISTED TISSUE ENGINEERING Article 02 April 2024 EFFECTS OF EXTRACELLULAR MATRIX VISCOELASTICITY ON CELLULAR BEHAVIOUR Article 26 August
2020 HUMAN INDUCED MESENCHYMAL STEM CELLS DISPLAY INCREASED SENSITIVITY TO MATRIX STIFFNESS Article Open access 19 May 2022 REFERENCES * Jaklenec, A., Stamp, A., Deweerd, E., Sherwin, A.
& Langer, R. Progress in the tissue engineering and stem cell industry “are we there yet?”. _Tissue Eng. B_ 18, 155–166 (2012). Article Google Scholar * McPherson, J. M. & Tubo, R.
Autologous chondrocyte transplantation (Carticel®): lessons learned and future challenges. _In Vitro Cell. Dev. Biol._ 40, 6A (2004). Google Scholar * Starzl, T. E. History of clinical
transplantation. _World J. Surg._ 24, 759–782 (2000). Article Google Scholar * Childs, P. G., Reid, S., Salmeron-Sanchez, M. & Dalby, M. J. Hurdles to uptake of mesenchymal stem cells
and their progenitors in therapeutic products. _Biochem. J._ 477, 3349–3366 (2020). Article Google Scholar * Yin, J. Q., Zhu, J. & Ankrum, J. A. Manufacturing of primed mesenchymal
stromal cells for therapy. _Nat. Biomed. Eng._ 3, 90–104 (2019). Article Google Scholar * Martins, J. P. et al. Towards an advanced therapy medicinal product based on mesenchymal stromal
cells isolated from the umbilical cord tissue: quality and safety data. _Stem Cell Res. Ther._ 5, 9 (2014). Article Google Scholar * Rozwadowska, N. et al. Optimization of human myoblasts
culture under different media conditions for application in the in vitro studies. _Am. J. Stem Cell_ 11, 1–11 (2022). Google Scholar * Sonnet, W. & Aznar‐López, C. Treatment of
delayed-union fractures of long bones with minimally invasive administration of allogeneic bone-forming cells differentiated from mesenchymal stem cells: a pilot clinical trial. _Bone
Innov._ 1, 3–7 (2019). Google Scholar * Czapla, J. et al. The effect of culture media on large-scale expansion and characteristic of adipose tissue-derived mesenchymal stromal cells. _Stem
Cell Res. Ther._ 10, 235 (2019). Article Google Scholar * Gualdi, T. et al. In vitro osteodifferentiation of intact human amniotic membrane is not beneficial in the context of bone repair.
_Cell Tissue Bank._ 20, 435–446 (2019). Article Google Scholar * Wang, N., Tytell, J. D. & Ingber, D. E. Mechanotransduction at a distance: mechanically coupling the extracellular
matrix with the nucleus. _Nat. Rev. Mol. Cell Biol._ 10, 75–82 (2009). Article Google Scholar * Vining, K. H. & Mooney, D. J. Mechanical forces direct stem cell behaviour in
development and regeneration. _Nat. Rev. Mol. Cell Biol._ 18, 728–742 (2017). Article Google Scholar * Wang, N. Review of cellular mechanotransduction. _J. Phys. D_ 50, 233002 (2017).
Article Google Scholar * Brusatin, G., Panciera, T., Gandin, A., Citron, A. & Piccolo, S. Biomaterials and engineered microenvironments to control YAP/TAZ-dependent cell behaviour.
_Nat. Mater._ 17, 1063–1075 (2018). Article Google Scholar * Melo-Fonseca, F. et al. Reengineering bone–implant interfaces for improved mechanotransduction and clinical outcomes. _Stem
Cell Rev. Rep._ 16, 1121–1138 (2020). Article Google Scholar * Discher, D. E., Janmey, P. & Wang, Y.-L. Tissue cells feel and respond to the stiffness of their substrate. _Science_
310, 1139–1143 (2005). Article Google Scholar * Cantini, M., Donnelly, H., Dalby, M. J. & Salmeron‐Sanchez, M. The plot thickens: the emerging role of matrix viscosity in cell
mechanotransduction. _Adv. Healthc. Mater._ 9, 1901259 (2020). Article Google Scholar * Luciano, M. et al. Cell monolayers sense curvature by exploiting active mechanics and nuclear
mechanoadaptation. _Nat. Phys._ 17, 1382–1390 (2021). Article Google Scholar * Roca-Cusachs, P., Conte, V. & Trepat, X. Quantifying forces in cell biology. _Nat. Cell Biol._ 19,
742–751 (2017). Article Google Scholar * Klumpers, D. D., Zhao, X., Mooney, D. J. & Smit, T. H. Cell mediated contraction in 3D cell-matrix constructs leads to spatially regulated
osteogenic differentiation. _Integr. Biol._ 5, 1174–1183 (2013). Article Google Scholar * Hodgkinson, T. et al. The use of nanovibration to discover specific and potent bioactive
metabolites that stimulate osteogenic differentiation in mesenchymal stem cells. _Sci. Adv._ 7, eabb7921 (2021). Article Google Scholar * Mirmalek-Sani, S. H. et al. Characterization and
multipotentiality of human fetal femur-derived cells: implications for skeletal tissue regeneration. _Stem Cell_ 24, 1042–1053 (2006). Article Google Scholar * Wu, J. et al. Joint
construction of micro-vibration stimulation and BCP scaffolds for enhanced bioactivity and self-adaptability tissue engineered bone grafts. _J. Mater. Chem. B_ 8, 4278–4288 (2020). Article
Google Scholar * ElDeeb, A. M. & Abdel-Aziem, A. A. Effect of whole-body vibration exercise on power profile and bone mineral density in postmenopausal women with osteoporosis: a
randomized controlled trial. _J. Manip. Physiol. Ther._ 43, 384–393 (2020). Article Google Scholar * Cooper, N. P., Vavakou, A. & van der Heijden, M. Vibration hotspots reveal
longitudinal funneling of sound-evoked motion in the mammalian cochlea. _Nat. Commun._ 9, 3054 (2018). Article Google Scholar * Brown, G. N., Sattler, R. L. & Guo, X. E. Experimental
studies of bone mechanoadaptation: bridging in vitro and in vivo studies with multiscale systems. _Interface Focus._ 6, 20150071 (2016). Article Google Scholar * Malone, A. M. et al.
Primary cilia mediate mechanosensing in bone cells by a calcium-independent mechanism. _Proc. Natl Acad. Sci. USA_ 104, 13325–13330 (2007). Article Google Scholar * Wang, J., Sun, Y.-X.
& Li, J. The role of mechanosensor Piezo1 in bone homeostasis and mechanobiology. _Dev. Biol._ 493, 80–88 (2022). Article Google Scholar * Vanmunster, M. et al. Mechanosensors control
skeletal muscle mass, molecular clocks, and metabolism. _Cell. Mol. Life Sci._ 79, 321 (2022). Article Google Scholar * Jaalouk, D. E. & Lammerding, J. Mechanotransduction gone awry.
_Nat. Rev. Mol. Cell Biol._ 10, 63–73 (2009). Article Google Scholar * Jiao, Y. et al. The crescendo pulse frequency of shear stress stimulates the endothelialization of bone marrow
mesenchymal stem cells on the luminal surface of decellularized scaffold in the bioreactor. _Bioengineered_ 13, 7925–7938 (2022). Article Google Scholar * Tan, Y. et al. Low-intensity
pulsed ultrasound stimulates proliferation of stem/progenitor cells: what we need to know to translate basic science research into clinical applications. _Asian J. Androl._ 23, 602 (2021).
Article Google Scholar * Markides, H. et al. Translation of remote control regenerative technologies for bone repair. _npj Regen. Med._ 3, 9 (2018). Article Google Scholar * Corrigan, M.
A. et al. TRPV4-mediates oscillatory fluid shear mechanotransduction in mesenchymal stem cells in part via the primary cilium. _Sci. Rep._ 8, 3824 (2018). Article Google Scholar * Hahn,
C. & Schwartz, M. A. Mechanotransduction in vascular physiology and atherogenesis. _Nat. Rev. Mol. Cell Biol._ 10, 53–62 (2009). Article Google Scholar * Dupont, S. et al. Role of
YAP/TAZ in mechanotransduction. _Nature_ 474, 179–183 (2011). Article Google Scholar * Beijer, N. R. et al. Dynamic adaptation of mesenchymal stem cell physiology upon exposure to surface
micropatterns. _Sci. Rep._ 9, 9099 (2019). Article Google Scholar * Pemberton, G. D. et al. Nanoscale stimulation of osteoblastogenesis from mesenchymal stem cells: nanotopography and
nanokicking. _Nanomedicine_ 10, 547–560 (2015). Article Google Scholar * Walther, B. K. et al. Mechanotransduction-on-chip: vessel-chip model of endothelial YAP mechanobiology reveals
matrix stiffness impedes shear response. _Lab Chip_ 21, 1738–1751 (2021). Article Google Scholar * Yarishkin, O. et al. Mechanotransduction and dynamic outflow regulation in trabecular
meshwork requires Piezo1 channels. Preprint at _bioRxiv_ https://doi.org/10.1101/2020.06.30.180653 (2020). * Melica, M. E. et al. Substrate stiffness modulates renal progenitor cell
properties via a ROCK-mediated mechanotransduction mechanism. _Cells_ 8, 1561 (2019). Article Google Scholar * Brown, T. D. Techniques for mechanical stimulation of cells in vitro: a
review. _J. Biomech._ 33, 3–14 (2000). Article Google Scholar * Stavenschi, E., Labour, M.-N. & Hoey, D. A. Oscillatory fluid flow induces the osteogenic lineage commitment of
mesenchymal stem cells: the effect of shear stress magnitude, frequency, and duration. _J. Biomech._ 55, 99–106 (2017). Article Google Scholar * Tsimbouri, P. M. et al. Stimulation of 3D
osteogenesis by mesenchymal stem cells using a nanovibrational bioreactor. _Nat. Biomed. Eng._ 1, 758–770 (2017). Article Google Scholar * Song, Z., Banks, R. W. & Bewick, G. S.
Modelling the mechanoreceptor’s dynamic behaviour. _J. Anat._ 227, 243–254 (2015). Article Google Scholar * Sawada, Y., Murase, M. & Sokabe, M. The gating mechanism of the bacterial
mechanosensitive channel MscL revealed by molecular dynamics simulations: from tension sensing to channel opening. _Channels_ 6, 317–331 (2012). Article Google Scholar * Appel, H. M. &
Cocroft, R. B. Plants respond to leaf vibrations caused by insect herbivore chewing. _Oecologia_ 175, 1257–1266 (2014). Article Google Scholar * von Muggenthaler, E. The felid purr: a
healing mechanism? _J. Acoust. Soc. Am._ 110, 2666–2666 (2001). Article Google Scholar * Nikukar, H. et al. Osteogenesis of mesenchymal stem cells by nanoscale mechanotransduction. _ACS
Nano_ 7, 2758–2767 (2013). Article Google Scholar * Yang, C., Tibbitt, M. W., Basta, L. & Anseth, K. S. Mechanical memory and dosing influence stem cell fate. _Nat. Mater._ 13, 645–652
(2014). Article Google Scholar * Thompson, M., Woods, K., Newberg, J., Oxford, J. T. & Uzer, G. Low-intensity vibration restores nuclear YAP levels and acute YAP nuclear shuttling in
mesenchymal stem cells subjected to simulated microgravity. _npj Microgravity_ 6, 35 (2020). Article Google Scholar * Kanchanawong, P. et al. Nanoscale architecture of integrin-based cell
adhesions. _Nature_ 468, 580–584 (2010). Article Google Scholar * Kilian, K. A., Bugarija, B., Lahn, B. T. & Mrksich, M. Geometric cues for directing the differentiation of mesenchymal
stem cells. _Proc. Natl Acad. Sci. USA_ 107, 4872–4877 (2010). Article Google Scholar * Lee, W. et al. The osteogenic differentiation of human dental pulp stem cells through G0/G1 arrest
and the p-ERK/Runx-2 pathway by sonic vibration. _Int. J. Mol. Sci._ 22, 10167 (2021). Article Google Scholar * Zhou, Y. et al. Osteogenic differentiation of bone marrow-derived
mesenchymal stromal cells on bone-derived scaffolds: effect of microvibration and role of ERK1/2 activation. _Eur. Cell Mater._ 22, 12–25 (2011). Article Google Scholar * Lu, Y. et al.
Vibration loading promotes osteogenic differentiation of bone marrow-derived mesenchymal stem cells via p38 MAPK signaling pathway. _J. Biomech._ 71, 67–75 (2018). Article Google Scholar *
Wu, R. W. et al. Piezoelectric microvibration mitigates estrogen loss-induced osteoporosis and promotes piezo1, microRNA-29a, and Wnt3a signaling in osteoblasts. _Int. J. Mol. Sci._ 22,
9476 (2021). Article Google Scholar * Bas, G. et al. Low intensity vibrations augment mesenchymal stem cell proliferation and differentiation capacity during in vitro expansion. _Sci.
Rep._ 10, 9369 (2020). Article Google Scholar * Halonen, H. T., Ihalainen, T. O., Hyväri, L., Miettinen, S. & Hyttinen, J. A. Cell adhesion and culture medium dependent changes in the
high frequency mechanical vibration induced proliferation, osteogenesis, and intracellular organization of human adipose stem cells. _J. Mech. Behav. Biomed. Mater._ 101, 103419 (2020).
Article Google Scholar * Hou, W., Zhang, D., Feng, X. & Zhou, Y. Low magnitude high frequency vibration promotes chondrogenic differentiation of bone marrow stem cells with involvement
of β-catenin signaling pathway. _Arch. Oral. Biol._ 118, 104860 (2020). Article Google Scholar * Safavi, A. S. et al. Efficacy of mechanical vibration in regulating mesenchymal stem cells
gene expression. _In Vitro Cell. Dev. Biol. Anim._ 55, 387–394 (2019). Article Google Scholar * Anggayasti, W. L., Imashiro, C., Kuribara, T., Totani, K. & Takemura, K. Low‐frequency
mechanical vibration induces apoptosis of A431 epidermoid carcinoma cells. _Eng. Life Sci._ 20, 232–238 (2020). Article Google Scholar * Zhao, Q., Lu, Y., Gan, X. & Yu, H. Low
magnitude high frequency vibration promotes adipogenic differentiation of bone marrow stem cells via P38 MAPK signal. _PLoS ONE_ 12, e0172954 (2017). Article Google Scholar *
Pongkitwitoon, S., Uzer, G., Rubin, J. & Judex, S. Cytoskeletal configuration modulates mechanically induced changes in mesenchymal stem cell osteogenesis, morphology, and stiffness.
_Sci. Rep._ 6, 34791 (2016). Article Google Scholar * Tapia-Rojo, R., Alonso-Caballero, Á. & Fernández, J. M. Talin folding as the tuning fork of cellular mechanotransduction. _Proc.
Natl Acad. Sci. USA_ 117, 21346–21353 (2020). Article Google Scholar * Griffin, X. L., Parsons, N., Costa, M. L. & Metcalfe, D. Ultrasound and shockwave therapy for acute fractures in
adults. _Cochrane Datab. Syst. Rev._ 3, CD008579 (2014). Google Scholar * Lam, T. et al. Effect of whole body vibration (WBV) therapy on bone density and bone quality in osteopenic girls
with adolescent idiopathic scoliosis: a randomized, controlled trial. _Osteopor. Int._ 24, 1623–1636 (2013). Article Google Scholar * Ambattu, L. A. & Yeo, L. Y. Sonomechanobiology:
vibrational stimulation of cells and its therapeutic implications. _Biophys. Rev._ 4, 021301 (2023). Article Google Scholar * Ling, L. et al. Low‐intensity pulsed ultrasound activates
ERK1/2 and PI3K‐Akt signalling pathways and promotes the proliferation of human amnion‐derived mesenchymal stem cells. _Cell Prolif._ 50, e12383 (2017). Article Google Scholar * Wang, Y.
et al. Low-intensity pulsed ultrasound promotes periodontal ligament stem cell migration through TWIST1-mediated SDF-1 expression. _Int. J. Mol. Med._ 42, 322–330 (2018); corrigendum 49, 38
(2022). * Wang, Y. et al. Study of bilineage differentiation of human-bone-marrow-derived mesenchymal stem cells in oxidized sodium alginate/_N_-succinyl chitosan hydrogels and synergistic
effects of RGD modification and low-intensity pulsed ultrasound. _Acta Biomater._ 10, 2518–2528 (2014). Article Google Scholar * Snehota, M., Vachutka, J., Ter Haar, G., Dolezal, L. &
Kolarova, H. Therapeutic ultrasound experiments in vitro: review of factors influencing outcomes and reproducibility. _Ultrasonics_ 107, 106167 (2020). Article Google Scholar * Gupta, D.
et al. Traditional multiwell plates and petri dishes limit the evaluation of the effects of ultrasound on cells in vitro. _Ultrasound Med. Biol._ 48, 1745–1761 (2022). Article Google
Scholar * Campsie, P. et al. Design, construction and characterisation of a novel nanovibrational bioreactor and cultureware for osteogenesis. _Sci. Rep._ 9, 12944 (2019). Article Google
Scholar * Prè, D. et al. High-frequency vibration treatment of human bone marrow stromal cells increases differentiation toward bone tissue. _Bone Marrow Res._ 2013, 803450 (2013). Article
Google Scholar * Uzer, G. et al. Separating fluid shear stress from acceleration during vibrations in vitro: identification of mechanical signals modulating the cellular response. _Cell.
Mol. Bioeng._ 5, 266–276 (2012). Article Google Scholar * Ito, Y. et al. Effects of vibration on differentiation of cultured PC12 cells. _Biotechnol. Bioeng._ 108, 592–599 (2011). Article
Google Scholar * Choi, S. & Kuchenbecker, K. J. Vibrotactile display: perception, technology, and applications. _Proc. IEEE_ 101, 2093–2104 (2012). Article Google Scholar * Dong, S.
Review on piezoelectric, ultrasonic, and magnetoelectric actuators. _J. Adv. Dielectr._ 2, 1230001 (2012). Article Google Scholar * Hensel, K., Mienkina, M. P. & Schmitz, G. Analysis
of ultrasound fields in cell culture wells for in vitro ultrasound therapy experiments. _Ultrasound Med. Biol._ 37, 2105–2115 (2011). Article Google Scholar * Patel, U. S. et al.
Ultrasound field characterization and bioeffects in multiwell culture plates. _J. Ther. Ultrasound_ 3, 8 (2015). Article Google Scholar * LaGier, A. J., Elbe, A., Thamke, A. &
Anderson, P. Vibration, a treatment for migraine, linked to calpain driven changes in actin cytoskeleton. _PLoS ONE_ 17, e0262058 (2022). Article Google Scholar * Tirkkonen, L. et al. The
effects of vibration loading on adipose stem cell number, viability and differentiation towards bone-forming cells. _J. R. Soc. Interf._ 8, 1736–1747 (2011). Article Google Scholar *
Bacabac, R. G. et al. Bone cell responses to high-frequency vibration stress: does the nucleus oscillate within the cytoplasm? _FASEB J._ 20, 858–864 (2006). Article Google Scholar * Prè,
D., Ceccarelli, G., Benedetti, L., Magenes, G. & De Angelis, M. G. Effects of low-amplitude, high-frequency vibrations on proliferation and differentiation of SAOS-2 human osteogenic
cell line. _Tissue Eng. C_ 15, 669–679 (2009). Article Google Scholar * Prè, D. et al. The differentiation of human adipose-derived stem cells (hASCs) into osteoblasts is promoted by low
amplitude, high frequency vibration treatment. _Bone_ 49, 295–303 (2011). Article Google Scholar * Nikukar, H. et al. Production of nanoscale vibration for stimulation of human mesenchymal
stem cells. _J. Biomed. Nanotechnol._ 12, 1478–1488 (2016). Article Google Scholar * Childs, P. G. et al. Use of nanoscale mechanical stimulation for control and manipulation of cell
behaviour. _Acta Biomater._ 34, 159–168 (2016). Article Google Scholar * Orapiriyakul, W. et al. Nanovibrational stimulation of mesenchymal stem cells induces therapeutic reactive oxygen
species and inflammation for three-dimensional bone tissue engineering. _ACS Nano_ 14, 10027–10044 (2020). Article Google Scholar * Abbott, B. P. et al. Observation of gravitational waves
from a binary black hole merger. _Phys. Rev. Lett._ 116, 061102 (2016). Article MathSciNet Google Scholar * Gao, H. et al. Low-level mechanical vibration enhances osteoblastogenesis via a
canonical Wnt signaling-associated mechanism. _Mol. Med. Rep._ 16, 317–324 (2017). Article Google Scholar * Enomoto, U., Imashiro, C. & Takemura, K. Collective cell migration of
fibroblasts is affected by horizontal vibration of the cell culture dish. _Eng. Life Sci._ 20, 402–411 (2020). Article Google Scholar * Mojena-Medina, D. et al. Design, implementation, and
validation of a piezoelectric device to study the effects of dynamic mechanical stimulation on cell proliferation, migration and morphology. _Sensors_ 20, 2155 (2020). Article Google
Scholar * Ota, T., Chiba, M. & Hayashi, H. Vibrational stimulation induces osteoblast differentiation and the upregulation of osteogenic gene expression in vitro. _Cytotechnology_ 68,
2287–2299 (2016). Article Google Scholar * Sancilio, S. et al. Effects of focused vibrations on human satellite cells. _Int. J. Mol. Sci._ 23, 6026 (2022). Article Google Scholar *
Takeuchi, R. et al. Effects of vibration and hyaluronic acid on activation of three-dimensional cultured chondrocytes. _Arthritis Rheum._ 54, 1897–1905 (2006). Article Google Scholar *
Grosman-Dziewiszek, P. et al. Influence of 40 Hz and 100 Hz vibration on SH-SY5Y cells growth and differentiation—a preliminary study. _Molecules_ 27, 3337 (2022). Article Google Scholar *
Lin, C. Y. et al. Yoda1 enhanced low-magnitude high-frequency vibration on osteocytes in regulation of MDA-MB-231 breast cancer cell migration. _Cancers_ 14, 3395 (2022). Article Google
Scholar * Uzer, G. et al. Cell mechanosensitivity to extremely low-magnitude signals is enabled by a LINCed nucleus. _Stem Cell_ 33, 2063–2076 (2015). Article Google Scholar * Lau, E. et
al. Effect of low-magnitude, high-frequency vibration on osteogenic differentiation of rat mesenchymal stromal cells. _J. Orthop. Res._ 29, 1075–1080 (2011). Article Google Scholar * Uzer,
G., Pongkitwitoon, S., Ete Chan, M. & Judex, S. Vibration induced osteogenic commitment of mesenchymal stem cells is enhanced by cytoskeletal remodeling but not fluid shear. _J.
Biomech._ 46, 2296–2302 (2013). Article Google Scholar * Lorusso, D., Nikolov, H. N., Holdsworth, D. W. & Dixon, S. J. Vibration of osteoblastic cells using a novel motion-control
platform does not acutely alter cytosolic calcium, but desensitizes subsequent responses to extracellular ATP. _J. Cell. Physiol._ 235, 5096–5110 (2020). Article Google Scholar * Coughlin,
T. R. & Niebur, G. L. Fluid shear stress in trabecular bone marrow due to low-magnitude high-frequency vibration. _J. Biomech._ 45, 2222–2229 (2012). Article Google Scholar * Ambattu,
L. A., Gelmi, A. & Yeo, L. Y. Short-duration high frequency megahertz-order nanomechanostimulation drives early and persistent osteogenic differentiation in mesenchymal stem cells.
_Small_ 18, 2106823 (2022). Article Google Scholar * Kennedy, J. W. et al. Nanovibrational stimulation inhibits osteoclastogenesis and enhances osteogenesis in co-cultures. _Sci. Rep._ 11,
22741 (2021). Article Google Scholar * Tong, Z., Duncan, R. & Jia, X. Modulating the behaviors of mesenchymal stem cells via the combination of high-frequency vibratory stimulations
and fibrous scaffolds. _Tissue Eng. A_ 19, 1862–1878 (2013). Article Google Scholar * Stein, G. S. & Lian, J. B. Molecular mechanisms mediating proliferation/differentiation
interrelationships during progressive development of the osteoblast phenotype. _Endocr. Rev._ 14, 424–442 (1993). Article Google Scholar * Yang, J. et al. Nanotopographical induction of
osteogenesis through adhesion, bone morphogenic protein cosignaling, and regulation of microRNAs. _ACS Nano_ 8, 9941–9953 (2014). Article Google Scholar * Li, Y. H. et al. Primary cilia
respond to intermittent low-magnitude, high-frequency vibration and mediate vibration-induced effects in osteoblasts. _Am. J. Physiol. Cell Physiol._ 318, C73–c82 (2020). Article Google
Scholar * Judex, S. & Pongkitwitoon, S. Differential efficacy of 2 vibrating orthodontic devices to alter the cellular response in osteoblasts, fibroblasts, and osteoclasts. _Dose
Response_ 16, 1559325818792112 (2018). Article Google Scholar * Marędziak, M., Lewandowski, D., Tomaszewski, K. A., Kubiak, K. & Marycz, K. The effect of low-magnitude low-frequency
vibrations (LMLF) on osteogenic differentiation potential of human adipose derived mesenchymal stem cells. _Cell Mol. Bioeng._ 10, 549–562 (2017). Article Google Scholar * Rosenberg, N.,
Levy, M. & Francis, M. Experimental model for stimulation of cultured human osteoblast-like cells by high frequency vibration. _Cytotechnology_ 39, 125–130 (2002). Article Google
Scholar * Marycz, K. et al. Low-frequency, low-magnitude vibrations (LFLM) enhances chondrogenic differentiation potential of human adipose derived mesenchymal stromal stem cells (hASCs).
_PeerJ_ 4, e1637 (2016). Article Google Scholar * Chen, X., He, F., Zhong, D.-Y. & Luo, Z.-P. Acoustic-frequency vibratory stimulation regulates the balance between osteogenesis and
adipogenesis of human bone marrow-derived mesenchymal stem cells. _Biomed. Res. Int._ 2015, 540731 (2015). Google Scholar * Baskan, O., Mese, G. & Ozcivici, E. Low-intensity vibrations
normalize adipogenesis-induced morphological and molecular changes of adult mesenchymal stem cells. _Proc. Inst. Mech. Eng. H_ 231, 160–168 (2017). Article Google Scholar * Baskan, O.,
Sarigil, O., Mese, G. & Ozcivici, E. Frequency-specific sensitivity of 3T3-L1 preadipocytes to low-intensity vibratory stimulus during adipogenesis. _In Vitro Cell. Dev. Biol. Anim._ 58,
452–461 (2022). Article Google Scholar * Cashion, A. T. et al. Programmable mechanobioreactor for exploration of the effects of periodic vibratory stimulus on mesenchymal stem cell
differentiation. _Biores. Open Access_ 3, 19–28 (2014). Article Google Scholar * Zhang, C. et al. Influence of different intensities of vibration on proliferation and differentiation of
human periodontal ligament stem cells. _Arch. Med. Sci._ 11, 638–646 (2015). Article Google Scholar * Lee, J., Abdeen, A. A., Tang, X., Saif, T. A. & Kilian, K. A. Geometric guidance
of integrin mediated traction stress during stem cell differentiation. _Biomaterials_ 69, 174–183 (2015). Article Google Scholar * Kilian, K. A. & Mrksich, M. Directing stem cell fate
by controlling the affinity and density of ligand-receptor interactions at the biomaterials interface. _Angew. Chem. Int. Edn_ 51, 4891–4895 (2012). Article Google Scholar * McBeath, R.,
Pirone, D. M., Nelson, C. M., Bhadriraju, K. & Chen, C. S. Cell shape, cytoskeletal tension, and RhoA regulate stem cell lineage commitment. _Dev. Cell_ 6, 483–495 (2004). Article
Google Scholar * Engler, A. J., Sen, S., Sweeney, H. L. & Discher, D. E. Matrix elasticity directs stem cell lineage specification. _Cell_ 126, 677–689 (2006). Article Google Scholar
* Ross, E. A. et al. Nanotopography reveals metabolites that maintain the immunomodulatory phenotype of mesenchymal stromal cells. _Nat. Commun._ 14, 753 (2023). Article Google Scholar *
Tsimbouri, P. M. et al. Using nanotopography and metabolomics to identify biochemical effectors of multipotency. _ACS Nano_ 6, 10239–10249 (2012). Article Google Scholar * Knight, C. G. et
al. The collagen-binding A-domains of integrins α1β1 and α2β1 recognize the same specific amino acid sequence, GFOGER, in native (triple-helical) collagens. _J. Biol. Chem._ 275, 35–40
(2000). Article Google Scholar * Dalby, M. J., Garcia, A. J. & Salmeron-Sanchez, M. Receptor control in mesenchymal stem cell engineering. _Nat. Rev. Mater_. 3, 17091 (2018). * Dalby,
M. J., Gadegaard, N. & Oreffo, R. O. Harnessing nanotopography and integrin-matrix interactions to influence stem cell fate. _Nat. Mater._ 13, 558–569 (2014). Article Google Scholar *
Elosegui-Artola, A. et al. Mechanical regulation of a molecular clutch defines force transmission and transduction in response to matrix rigidity. _Nat. Cell Biol._ 18, 540–548 (2016).
Article Google Scholar * Bennett, M. et al. Molecular clutch drives cell response to surface viscosity. _Proc. Natl Acad. Sci. USA_ 115, 1192–1197 (2018). Article Google Scholar *
Malmstrom, J. et al. Focal complex maturation and bridging on 200 nm vitronectin but not fibronectin patches reveal different mechanisms of focal adhesion formation. _Nano Lett._ 11,
2264–2271 (2011). Article Google Scholar * Elosegui-Artola, A. et al. Force triggers YAP nuclear entry by regulating transport across nuclear pores. _Cell_ 171, 1397–1410.e14 (2017).
Article Google Scholar * Kim, J.-M. et al. The ERK MAPK pathway is essential for skeletal development and homeostasis. _Int. J. Mol. Sci._ 20, 1803 (2019). Article Google Scholar * Ge,
C. et al. Reciprocal control of osteogenic and adipogenic differentiation by ERK/MAP kinase phosphorylation of Runx2 and PPARγ transcription factors. _J. Cell Physiol._ 231, 587–596 (2016).
Article Google Scholar * Chen, Y. et al. Beta-catenin signaling pathway is crucial for bone morphogenetic protein 2 to induce new bone formation. _J. Biol. Chem._ 282, 526–533 (2007).
Article Google Scholar * Hou, W. W., Zhu, Z. L., Zhou, Y., Zhang, C. X. & Yu, H. Y. Involvement of Wnt activation in the micromechanical vibration-enhanced osteogenic response of
osteoblasts. _J. Orthopaedic Sci._ 16, 598–605 (2011). Article Google Scholar * Miyazaki, A. et al. Coordination of WNT signaling and ciliogenesis during odontogenesis by piezo type
mechanosensitive ion channel component 1. _Sci. Rep._ 9, 14762 (2019). Article Google Scholar * Liu, Y. et al. Hydrogen sulfide maintains mesenchymal stem cell function and bone
homeostasis via regulation of Ca2+ channel sulfhydration. _Cell Stem Cell_ 15, 66–78 (2014). Article Google Scholar * Gaur, T. et al. Canonical WNT signaling promotes osteogenesis by
directly stimulating Runx2 gene expression. _J. Biol. Chem._ 280, 33132–33140 (2005). Article Google Scholar * Choi, Y. et al. Sound waves induce neural differentiation of human bone
marrow-derived mesenchymal stem cells via ryanodine receptor-induced calcium release and Pyk2 activation. _Appl. Biochem. Biotechnol._ 180, 682–694 (2016). Article Google Scholar * Coste,
B. et al. Piezo proteins are pore-forming subunits of mechanically activated channels. _Nature_ 483, 176–181 (2012). Article Google Scholar * Kefauver, J. M., Ward, A. B. &
Patapoutian, A. Discoveries in structure and physiology of mechanically activated ion channels. _Nature_ 587, 567–576 (2020). Article Google Scholar * Li, Y. H. et al. Crosstalk between
the COX2–PGE2–EP4 signaling pathway and primary cilia in osteoblasts after mechanical stimulation. _J. Cell Physiol._ 236, 4764–4777 (2021). Article Google Scholar * Loi, F. et al.
Inflammation, fracture and bone repair. _Bone_ 86, 119–130 (2016). Article Google Scholar * Ranzinger, J. et al. Nanoscale arrangement of apoptotic ligands reveals a demand for a minimal
lateral distance for efficient death receptor activation. _Nano Lett._ 9, 4240–4245 (2009). Article Google Scholar * Varum, S. et al. Energy metabolism in human pluripotent stem cells and
their differentiated counterparts. _PLoS ONE_ 6, e20914 (2011). Article Google Scholar * Varum, S. et al. Enhancement of human embryonic stem cell pluripotency through inhibition of the
mitochondrial respiratory chain. _Stem Cell Res._ 3, 142–156 (2009). Article Google Scholar * Marín-Cascales, E. et al. Whole-body vibration training and bone health in postmenopausal
women: a systematic review and meta-analysis. _Medicine_ 97, e11918 (2018). Article Google Scholar * Leighton, R., Phillips, M., Bhandari, M. & Zura, R. Low intensity pulsed ultrasound
(LIPUS) use for the management of instrumented, infected, and fragility non-unions: a systematic review and meta-analysis of healing proportions. _BMC Musculoskelet. Disord._ 22, 1–9
(2021). Article Google Scholar * Auersperg, V. & Trieb, K. Extracorporeal shock wave therapy: an update. _EFORT Open. Rev._ 5, 584–592 (2020). Article Google Scholar * Köllmer, M.,
Buhrman, J. S., Zhang, Y. & Gemeinhart, R. A. Markers are shared between adipogenic and osteogenic differentiated mesenchymal stem cells. _J. Dev. Biol. Tissue Eng._ 5, 18 (2013).
Article Google Scholar * Pel, J. et al. Platform accelerations of three different whole-body vibration devices and the transmission of vertical vibrations to the lower limbs. _Med. Eng.
Phys._ 31, 937–944 (2009). Article Google Scholar * Rehn, B., Lidström, J., Skoglund, J. & Lindström, B. Effects on leg muscular performance from whole‐body vibration exercise: a
systematic review. _Scand. J. Med. Sci. Sports_ 17, 2–11 (2007). Article Google Scholar * Sitjà-Rabert, M. et al. Efficacy of whole body vibration exercise in older people: a systematic
review. _Disabil. Rehabil._ 34, 883–893 (2012). Article Google Scholar * Chanou, K., Gerodimos, V., Karatrantou, K. & Jamurtas, A. Whole-body vibration and rehabilitation of chronic
diseases: a review of the literature. _J. Sports Sci. Med._ 11, 187 (2012). Google Scholar * Cardinale, M. & Wakeling, J. Whole body vibration exercise: are vibrations good for you?
_Br. J. Sports Med._ 39, 585–589 (2005). Article Google Scholar * Fischer, M. et al. Long-term effects of whole-body vibration on human gait: a systematic review and meta-analysis. _Front.
Neurol._ 10, 627 (2019). Article Google Scholar * Slatkovska, L., Alibhai, S., Beyene, J. & Cheung, A. Effect of whole-body vibration on BMD: a systematic review and meta-analysis.
_Osteoporos. Int._ 21, 1969–1980 (2010). Article Google Scholar * DadeMatthews, O. O. et al. Systematic review and meta-analyses on the effects of whole-body vibration on bone health.
_Complement. Ther. Med._ 65, 102811 (2022). Article Google Scholar * Peretti, A. L., Ciqueleiro, R. T., Flores, L. J. F. & Bertolini, G. R. F. Use of whole-body vibration as
osteoporosis treatment in postmenopausal women: a systematic review. _Eur. J. Clin. Exp. Med_. 17, 146–152 (2019). Article Google Scholar * Leung, K. S. et al. Low‐magnitude high‐frequency
vibration accelerates callus formation, mineralization, and fracture healing in rats. _J. Orthop. Res._ 27, 458–465 (2009). Article Google Scholar * Shi, H.-F., Cheung, W.-H., Qin, L.,
Leung, A. H.-C. & Leung, K.-S. Low-magnitude high-frequency vibration treatment augments fracture healing in ovariectomy-induced osteoporotic bone. _Bone_ 46, 1299–1305 (2010). Article
Google Scholar * Wong, R. M. Y. et al. Fibrinolysis as a target to enhance osteoporotic fracture healing by vibration therapy in a metaphyseal fracture model. _Bone Jt Res._ 10, 41–50
(2021). Article Google Scholar * Chow, S. et al. Mechanical stimulation enhanced estrogen receptor expression and callus formation in diaphyseal long bone fracture healing in
ovariectomy-induced osteoporotic rats. _Osteoporos. Int._ 27, 2989–3000 (2016). Article Google Scholar * Haffner-Luntzer, M., Lackner, I., Liedert, A., Fischer, V. & Ignatius, A.
Effects of low-magnitude high-frequency vibration on osteoblasts are dependent on estrogen receptor α signaling and cytoskeletal remodeling. _Biochem. Biophys. Res. Commun._ 503, 2678–2684
(2018). Article Google Scholar * Chow, S. et al. Vibration treatment modulates macrophage polarisation and enhances early inflammatory response in oestrogen-deficient osteoporotic-fracture
healing. _Eur. Cell Mater._ 38, 228–245 (2019). Article Google Scholar * Jawed, Y., Beli, E., March, K., Kaleth, A. & Loghmani, M. T. Whole-body vibration training increases
stem/progenitor cell circulation levels and may attenuate inflammation. _Military Med._ 185, 404–412 (2020). Article Google Scholar * Jing, D. et al. Effect of low-level mechanical
vibration on osteogenesis and osseointegration of porous titanium implants in the repair of long bone defects. _Sci. Rep._ 5, 17134 (2015). Article Google Scholar * Wang, J. et al.
Vibration and β‐hydroxy‐β‐methylbutyrate treatment suppresses intramuscular fat infiltration and adipogenic differentiation in sarcopenic mice. _J. Cachexia, Sarcopenia Muscle_ 11, 564–577
(2020). Article Google Scholar * Judex, S. & Rubin, C. Is bone formation induced by high-frequency mechanical signals modulated by muscle activity? _J. Musculoskelet. Neuronal
Interact._ 10, 3 (2010). Google Scholar * Seo, B. R. et al. Skeletal muscle regeneration with robotic actuation–mediated clearance of neutrophils. _Sci. Transl. Med._ 13, eabe8868 (2021).
Article Google Scholar * Lara-Castillo, N. et al. In vivo mechanical loading rapidly activates β-catenin signaling in osteocytes through a prostaglandin mediated mechanism. _Bone_ 76,
58–66 (2015). Article Google Scholar * Liu, C. et al. Effects of mechanical loading on cortical defect repair using a novel mechanobiological model of bone healing. _Bone_ 108, 145–155
(2018). Article Google Scholar * Birmingham, E. et al. Mechanical stimulation of bone marrow in situ induces bone formation in trabecular explants. _Ann. Biomed. Eng._ 43, 1036–1050
(2015). Article Google Scholar * Zhao, C., Liu, H., Tian, C., Zhang, C. & Wang, W. Multi-scale numerical simulation on mechano-transduction of osteocytes in different gravity fields.
_Comput. Meth. Biomech. Biomed. Eng._ 26, 1419–1430 (2023). Article Google Scholar * Williams, J. A. et al. Developing and investigating a nanovibration intervention for the
prevention/reversal of bone loss following spinal cord injury. _ACS Nano_ 18, 17630–17641 (2024). Article Google Scholar * McKnight, C. L., Doman, D. A., Brown, J. A., Bance, M. &
Adamson, R. B. Direct measurement of the wavelength of sound waves in the human skull. _J. Acoust. Soc. Am._ 133, 136–145 (2013). Article Google Scholar * McLeod, R., Roberts, W., Perry,
I., Richardson, B. & Culling, J. Scanning laser Doppler vibrometry of the cranium when stimulated by a B71 bone transducer. _Appl. Acoust._ 142, 53–58 (2018). Article Google Scholar *
Dobrev, I. et al. Sound wave propagation on the human skull surface with bone conduction stimulation. _Hearing Res._ 355, 1–13 (2017). Article Google Scholar * Busse, J. W. et al.
Re-evaluation of low intensity pulsed ultrasound in treatment of tibial fractures (TRUST): randomized clinical trial. _BMJ_ 355, i5351 (2016). Google Scholar * Poolman, R. W. et al. Low
intensity pulsed ultrasound (LIPUS) for bone healing: a clinical practice guideline. _BMJ_ 356, j576 (2017). Article Google Scholar * Dolgin, E. Sizzling interest in lab-grown meat belies
lack of basic research. _Nature_ 566, 161–163 (2019). Article Google Scholar * Stout, A. J. et al. Simple and effective serum-free medium for sustained expansion of bovine satellite cells
for cell cultured meat. _Commun. Biol._ 5, 466 (2022). Article Google Scholar * Coords, M. et al. The effects of low-intensity pulsed ultrasound upon diabetic fracture healing. _J. Orthop.
Res._ 29, 181–188 (2011). Article Google Scholar * Curtis, A. S. et al. Cell interactions at the nanoscale: piezoelectric stimulation. _IEEE Trans. Nanobiosci._ 12, 247–254 (2013).
Article Google Scholar * Karimi, E. et al. Nanoscale vibration could promote tenogenic differentiation of umbilical cord mesenchymal stem cells. _In Vitro Cell. Dev. Biol. Anim._ 59,
401–409 (2023). Article Google Scholar * Kim, I. S., Song, Y. M., Lee, B. & Hwang, S. J. Human mesenchymal stromal cells are mechanosensitive to vibration stimuli. _J. Dent. Res._ 91,
1135–1140 (2012). Article Google Scholar * Demiray, L. & Ozcivici, E. Bone marrow stem cells adapt to low-magnitude vibrations by altering their cytoskeleton during quiescence and
osteogenesis. _Turk. J. Biol._ 39, 88–97 (2015). Article Google Scholar * Pravitharangul, A., Suttapreyasri, S. & Leethanakul, C. Iliac and mandible osteoblasts exhibit varied
responses to LMHF vibration. _Cell Biol. Int._ 42, 1349–1357 (2018). Article Google Scholar * Chen, B. et al. Low-magnitude, high-frequency vibration promotes the adhesion and the
osteogenic differentiation of bone marrow-derived mesenchymal stem cells cultured on a hydroxyapatite-coated surface: The direct role of Wnt/β-catenin signaling pathway activation. _Int. J.
Mol. Med._ 38, 1531–1540 (2016). Article Google Scholar * Macione, J. et al. Stimulation of osteoblast differentiation with guided ultrasound waves. _J. Ther. Ultrasound_ 3, 12 (2015).
Article Google Scholar * Chu, Y. C., Lim, J., Hwang, W. H., Lin, Y. X. & Wang, J. L. Piezoelectric stimulation by ultrasound facilitates chondrogenesis of mesenchymal stem cells. _J.
Acoust. Soc. Am._ 148, El58 (2020). Article Google Scholar * Hortobagyi, D. et al. In vitro mechanical vibration down-regulates pro-inflammatory and pro-fibrotic signaling in human vocal
fold fibroblasts. _PLoS ONE_ 15, e0241901 (2020). Article Google Scholar * García-López, S., Villanueva, R. E., Massó-Rojas, F., Páez-Arenas, A. & Meikle, M. C. Micro-vibrations at 30
Hz on bone cells cultivated in vitro produce soluble factors for osteoclast inhibition and osteoblast activity. _Arch. Oral. Biol._ 110, 104594 (2020). Article Google Scholar * Sun, T. et
al. Effects of mechanical vibration on cell morphology, proliferation, apoptosis, and cytokine expression/secretion in osteocyte-like MLO-Y4 cells exposed to high glucose. _Cell Biol. Int._
44, 216–228 (2019). Article Google Scholar * Wang, C.-Z. et al. Low-magnitude vertical vibration enhances myotube formation in C2C12 myoblasts. _J. Appl. Physiol._ 109, 840–848 (2010).
Article Google Scholar * Lin, Y. H. et al. The essential role of stathmin in myoblast C2C12 for vertical vibration-induced myotube formation. _Biomolecules_ 11, 1583 (2021). Article
Google Scholar * Choi, Y. K., Cho, H., Seo, Y. K., Yoon, H. H. & Park, J. K. Stimulation of sub-sonic vibration promotes the differentiation of adipose tissue-derived mesenchymal stem
cells into neural cells. _Life Sci._ 91, 329–337 (2012). Article Google Scholar * Cho, H., Seo, Y.-K., Yoon, H.-H., Choi, Y.-K. & Park, J.-K. Neural differentiation of umbilical cord
mesenchymal stem cells by sub-sonic vibration. _Life Sci._ 90, 591–599 (2012). Article Google Scholar * Cho, H., Park, H. J. & Seo, Y. K. Induction of _PLXNA4_ gene during neural
differentiation in human umbilical-cord-derived mesenchymal stem cells by low-intensity sub-sonic vibration. _Int. J. Mol. Sci._ 23, 1522 (2022). Article Google Scholar * Benjakul, S.,
Leethanakul, C. & Jitpukdeebodintra, S. Low magnitude high frequency vibration induces RANKL via cyclooxygenase pathway in human periodontal ligament cells in vitro. _J. Oral. Biol.
Craniofac. Res._ 9, 251–255 (2019). Article Google Scholar * Ye, M. et al. Vibration induces BAFF overexpression and aberrant O-glycosylation of IgA1 in cultured human tonsillar
mononuclear cells in IgA nephropathy. _Biomed. Res. Int._ 2016, 9125960 (2016). Article Google Scholar * Touchstone, H. et al. Recovery of stem cell proliferation by low intensity
vibration under simulated microgravity requires LINC complex. _npj Microgravity_ 5, 11 (2019). Article Google Scholar * Robertson, S. N. et al. Reduction of _Pseudomonas aeruginosa_
biofilm formation through the application of nanoscale vibration. _J. Biosci. Bioeng._ 129, 379–386 (2020). Article Google Scholar * Tanaka, S. M. et al. Effects of broad frequency
vibration on cultured osteoblasts. _J. Biomech._ 36, 73–80 (2003). Article Google Scholar * Ballikaya, S. et al. Process data of allogeneic ex vivo-expanded ABCB5+ mesenchymal stromal
cells for human use: off-the-shelf GMP-manufactured donor-independent ATMP. _Stem Cell Res. Ther._ 11, 482 (2020). Article Google Scholar * Simaria, A. S. et al. Allogeneic cell therapy
bioprocess economics and optimization: single‐use cell expansion technologies. _Biotechnol. Bioeng._ 111, 69–83 (2014). Article Google Scholar * Lawson, T. et al. Process development for
expansion of human mesenchymal stromal cells in a 50L single-use stirred tank bioreactor. _Biochem. Eng. J._ 120, 49–62 (2017). Article Google Scholar * Karnieli, O. et al. A consensus
introduction to serum replacements and serum-free media for cellular therapies. _Cytotherapy_ 19, 155–169 (2017). Article Google Scholar Download references ACKNOWLEDGEMENTS We thank EPSRC
for grants EP/N013905/1 and EP/P001114/1. AUTHOR INFORMATION AUTHORS AND AFFILIATIONS * Centre for the Cellular Microenvironment (CeMi), Department of Biomedical Engineering, University of
Strathclyde, Glasgow, UK Olivia Johnson-Love, Stuart Reid & Peter G. Childs * Centre for the Cellular Microenvironment (CeMi), University of Glasgow, Glasgow, UK Manuel Salmeron-Sanchez
& Matthew J. Dalby Authors * Olivia Johnson-Love View author publications You can also search for this author inPubMed Google Scholar * Manuel Salmeron-Sanchez View author publications
You can also search for this author inPubMed Google Scholar * Stuart Reid View author publications You can also search for this author inPubMed Google Scholar * Peter G. Childs View author
publications You can also search for this author inPubMed Google Scholar * Matthew J. Dalby View author publications You can also search for this author inPubMed Google Scholar CONTRIBUTIONS
O.J.-L., P.G.C. and M.J.D. led the writing of the review, with all authors involved in contributing to different sections. All authors critically read the review. CORRESPONDING AUTHORS
Correspondence to Peter G. Childs or Matthew J. Dalby. ETHICS DECLARATIONS COMPETING INTERESTS The authors declare no competing interests. PEER REVIEW PEER REVIEW INFORMATION _Nature Reviews
Bioengineering_ thanks Benoit Ladoux, Sylvain Gabriele, Marine Luciano and the other, anonymous, reviewer(s) for their contribution to the peer review of this work. ADDITIONAL INFORMATION
PUBLISHER’S NOTE Springer Nature remains neutral with regard to jurisdictional claims in published maps and institutional affiliations. RELATED LINKS THE FAILURE OF A PHASE IIB TRIAL OF
ALLOB: https://www.fiercebiotech.com/biotech/bone-therapeutics-final-asset-buried-after-failure-phase-2-fracture-study RIGHTS AND PERMISSIONS Springer Nature or its licensor (e.g. a society
or other partner) holds exclusive rights to this article under a publishing agreement with the author(s) or other rightsholder(s); author self-archiving of the accepted manuscript version of
this article is solely governed by the terms of such publishing agreement and applicable law. Reprints and permissions ABOUT THIS ARTICLE CITE THIS ARTICLE Johnson-Love, O.,
Salmeron-Sanchez, M., Reid, S. _et al._ Vibration-based cell engineering. _Nat Rev Bioeng_ 3, 408–429 (2025). https://doi.org/10.1038/s44222-025-00273-x Download citation * Published: 03
February 2025 * Issue Date: May 2025 * DOI: https://doi.org/10.1038/s44222-025-00273-x SHARE THIS ARTICLE Anyone you share the following link with will be able to read this content: Get
shareable link Sorry, a shareable link is not currently available for this article. Copy to clipboard Provided by the Springer Nature SharedIt content-sharing initiative