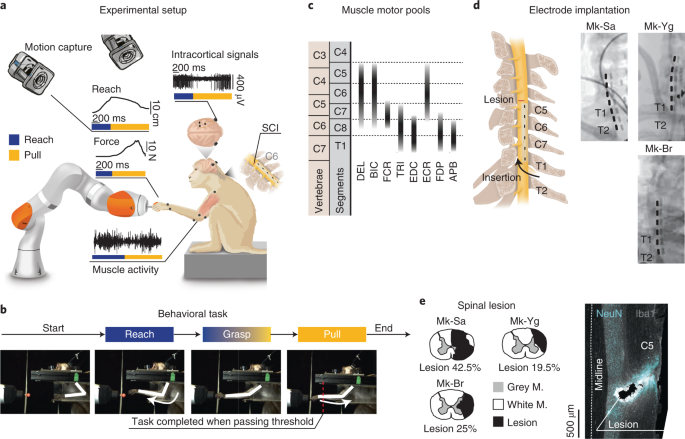
- Select a language for the TTS:
- UK English Female
- UK English Male
- US English Female
- US English Male
- Australian Female
- Australian Male
- Language selected: (auto detect) - EN
Play all audios:
ABSTRACT Regaining arm control is a top priority for people with paralysis. Unfortunately, the complexity of the neural mechanisms underlying arm control has limited the effectiveness of
neurotechnology approaches. Here, we exploited the neural function of surviving spinal circuits to restore voluntary arm and hand control in three monkeys with spinal cord injury, using
spinal cord stimulation. Our neural interface leverages the functional organization of the dorsal roots to convey artificial excitation via electrical stimulation to relevant spinal segments
at appropriate movement phases. Stimulation bursts targeting specific spinal segments produced sustained arm movements, enabling monkeys with arm paralysis to perform an unconstrained
reach-and-grasp task. Stimulation specifically improved strength, task performances and movement quality. Electrophysiology suggested that residual descending inputs were necessary to
produce coordinated movements. The efficacy and reliability of our approach hold realistic promises of clinical translation. Access through your institution Buy or subscribe This is a
preview of subscription content, access via your institution ACCESS OPTIONS Access through your institution Access Nature and 54 other Nature Portfolio journals Get Nature+, our best-value
online-access subscription $29.99 / 30 days cancel any time Learn more Subscribe to this journal Receive 12 print issues and online access $209.00 per year only $17.42 per issue Learn more
Buy this article * Purchase on SpringerLink * Instant access to full article PDF Buy now Prices may be subject to local taxes which are calculated during checkout ADDITIONAL ACCESS OPTIONS:
* Log in * Learn about institutional subscriptions * Read our FAQs * Contact customer support SIMILAR CONTENT BEING VIEWED BY OTHERS EPIDURAL STIMULATION OF THE CERVICAL SPINAL CORD FOR
POST-STROKE UPPER-LIMB PARESIS Article 20 February 2023 RECRUITMENT OF UPPER-LIMB MOTONEURONS WITH EPIDURAL ELECTRICAL STIMULATION OF THE CERVICAL SPINAL CORD Article Open access 19 January
2021 ACTIVITY-DEPENDENT SPINAL CORD NEUROMODULATION RAPIDLY RESTORES TRUNK AND LEG MOTOR FUNCTIONS AFTER COMPLETE PARALYSIS Article 07 February 2022 DATA AVAILABILITY Due to the sensitive
nature of the dataset, which contains graphic information on monkeys, raw data, including videos, will be available upon reasonable request to the corresponding author and after
authorization from the Swiss cantonal authorities. A set of preprocessed data will be deposited on the Open-Data Commons for Spinal Cord Injury (https://odc-sci.org). Source data are
provided with this paper. CODE AVAILABILITY Software routines utilized for data analysis will be deposited on GitHub under search keyword NN-A75365C. REFERENCES * ICCP. International
Campaign for Cures of Spinal Cord Injury Paralysis. http://www.campaignforcure.org * _Stroke Facts_ (National Center for Chronic Disease Prevention and Health Promotion, Division for Heart
Disease and Stroke. Stroke facts, 2020); https://www.cdc.gov/stroke/facts.htm * Anderson, K. D. Targeting recovery: priorities of the spinal cord-injured population. _J. Neurotrauma_ 21,
1371–1383 (2004). Article PubMed Google Scholar * Moreland, J. D. et al. Needs assessment of individuals with stroke after discharge from hospital stratified by acute Functional
Independence Measure score. _Disabil. Rehabil._ 31, 2185–2195 (2009). Article PubMed Google Scholar * Lemon, R. N. Descending pathways in motor control. _Annu. Rev. Neurosci._ 31, 195–218
(2008). Article CAS PubMed Google Scholar * Griffin, D. M. & Strick, P. L. The motor cortex uses active suppression to sculpt movement. _Sci. Adv._ 6, eabb8395 (2020). Article CAS
PubMed PubMed Central Google Scholar * Seki, K., Perlmutter, S. I. & Fetz, E. E. Sensory input to primate spinal cord is presynaptically inhibited during voluntary movement. _Nat.
Neurosci._ 6, 1309–1316 (2003). Article CAS PubMed Google Scholar * Lebedev, M. A. & Nicolelis, M. A. Brain-machine interfaces: from basic science to neuroprostheses and
neurorehabilitation. _Physiol. Rev._ 97, 767–837 (2017). Article PubMed Google Scholar * Nishimura, Y., Perlmutter, S. I. & Fetz, E. E. Restoration of upper limb movement via
artificial corticospinal and musculospinal connections in a monkey with spinal cord injury. _Front. Neural Circuits_ 7, 57 (2013). Article PubMed PubMed Central Google Scholar *
Shanechi, M. M., Hu, R. C. & Williams, Z. M. A cortical-spinal prosthesis for targeted limb movement in paralysed primate avatars. _Nat. Commun._ 5, 3237 (2014). Article PubMed CAS
Google Scholar * Zimmermann, J. B. & Jackson, A. Closed-loop control of spinal cord stimulation to restore hand function after paralysis. _Front. Neurosci._ 8, 87 (2014). Article
PubMed PubMed Central Google Scholar * Ethier, C., Oby, E. R., Bauman, M. J. & Miller, L. E. Restoration of grasp following paralysis through brain-controlled stimulation of muscles.
_Nature_ 485, 368–371 (2012). Article CAS PubMed PubMed Central Google Scholar * Bouton, C. E. et al. Restoring cortical control of functional movement in a human with quadriplegia.
_Nature_ https://doi.org/10.1038/nature17435 (2016). * Ajiboye, A. B. et al. Restoration of reaching and grasping movements through brain-controlled muscle stimulation in a person with
tetraplegia: a proof-of-concept demonstration. _Lancet_ https://doi.org/10.1016/S0140-6736(17)30601-3 (2017). * Giat, Y., Mizrahi, J. & Levy, M. A musculotendon model of the fatigue
profiles of paralyzed quadriceps muscle under FES. _IEEE Trans. Biomed. Eng._ 40, 664–674 (1993). Article CAS PubMed Google Scholar * Edgerton, V. R. et al. Training locomotor networks.
_Brain Res. Rev._ 57, 241–254 (2008). Article PubMed Google Scholar * Holinski, B. J. et al. Intraspinal microstimulation produces over-ground walking in anesthetized cats. _J. Neural
Eng._ 13, 056016 (2016). Article CAS PubMed PubMed Central Google Scholar * Capogrosso, M. et al. A computational model for epidural electrical stimulation of spinal sensorimotor
circuits. _J. Neurosci._ 33, 19326–19340 (2013). Article CAS PubMed PubMed Central Google Scholar * Formento, E. et al. Electrical spinal cord stimulation must preserve proprioception
to enable locomotion in humans with spinal cord injury. _Nat. Neurosci._ 21, 1728–1741 (2018). Article CAS PubMed PubMed Central Google Scholar * Wagner, F. B. et al. Targeted
neurotechnology restores walking in humans with spinal cord injury. _Nature_ 563, 65–71 (2018). Article CAS PubMed Google Scholar * Angeli, C. A., Edgerton, V. R., Gerasimenko, Y. P.
& Harkema, S. J. Altering spinal cord excitability enables voluntary movements after chronic complete paralysis in humans. _Brain_ 137, 1394–1409 (2014). Article PubMed PubMed Central
Google Scholar * Ichiyama, R. M., Gerasimenko, Y. P., Zhong, H., Roy, R. R. & Edgerton, V. R. Hindlimb stepping movements in complete spinal rats induced by epidural spinal cord
stimulation. _Neurosci. Lett._ 383, 339–344 (2005). Article CAS PubMed Google Scholar * Courtine, G. et al. Transformation of nonfunctional spinal circuits into functional states after
the loss of brain input. _Nat. Neurosci._ 12, 1333–1342 (2009). Article CAS PubMed PubMed Central Google Scholar * van den Brand, R. et al. Restoring voluntary control of locomotion
after paralyzing spinal cord injury. _Science_ 336, 1182–1185 (2012). Article PubMed CAS Google Scholar * Grahn, P. J. et al. Enabling task-specific volitional motor functions via spinal
cord neuromodulation in a human with paraplegia. _Mayo Clin. Proc._ 92, 544–554 (2017). Article PubMed Google Scholar * Angeli, C. A. et al. Recovery of over-ground walking after chronic
motor complete spinal cord injury. _N. Engl. J. Med._ 379, 1244–1250 (2018). Article PubMed Google Scholar * Gill, M. L. et al. Neuromodulation of lumbosacral spinal networks enables
independent stepping after complete paraplegia. _Nat. Med_. https://doi.org/10.1038/s41591-018-0175-7 (2018). * Alam, M. et al. Evaluation of optimal electrode configurations for epidural
spinal cord stimulation in cervical spinal cord injured rats. _J. Neurosci. Methods_ 247, 50–57 (2015). Article PubMed PubMed Central Google Scholar * Lu, D. C. et al. Engaging cervical
spinal cord networks to reenable volitional control of hand function in tetraplegic patients. _Neurorehabil. Neural Repair_ 30, 951–962 (2016). Article PubMed PubMed Central Google
Scholar * Inanici, F., Brighton, L. N., Samejima, S., Hofstetter, C. P. & Moritz, C. T. Transcutaneous spinal cord stimulation restores hand and arm function after spinal cord injury.
_IEEE Trans. Neural Syst. Rehabil. Eng_. https://doi.org/10.1109/TNSRE.2021.3049133 (2021). * Kapadia, N., Zivanovic, V. & Popovic, M. Restoring voluntary grasping function in
individuals with incomplete chronic spinal cord injury: pilot study. _Top. Spinal Cord. Inj. Rehabil._ 19, 279–287 (2013). Article PubMed PubMed Central Google Scholar * Grillner, S. The
motor infrastructure: from ion channels to neuronal networks. _Nat. Rev. Neurosci._ 4, 573–586 (2003). Article CAS PubMed Google Scholar * Giszter, S. F. Motor primitives—new data and
future questions. _Curr. Opin. Neurobiol._ 33, 156–165 (2015). Article CAS PubMed PubMed Central Google Scholar * Lemon, R. N. & Griffiths, J. Comparing the function of the
corticospinal system in different species: organizational differences for motor specialization? _Muscle Nerve_ 32, 261–279 (2005). Article PubMed Google Scholar * Kinoshita, M. et al.
Genetic dissection of the circuit for hand dexterity in primates. _Nature_ 487, 235–238 (2012). Article CAS PubMed Google Scholar * Weiler, J., Gribble, P. L. & Pruszynski, J. A.
Spinal stretch reflexes support efficient hand control. _Nat. Neurosci._ 22, 529–533 (2019). Article CAS PubMed Google Scholar * Sauerbrei, B. A. et al. Cortical pattern generation
during dexterous movement is input-driven. _Nature_ 577, 386–391 (2020). Article CAS PubMed Google Scholar * Capogrosso, M. et al. Configuration of electrical spinal cord stimulation
through real-time processing of gait kinematics. _Nat. Protoc_. https://doi.org/10.1038/s41596-018-0030-9 (2018). * Capogrosso, M. et al. A brain–spine interface alleviating gait deficits
after spinal cord injury in primates. _Nature_ 539, 284–288 (2016). Article PubMed PubMed Central Google Scholar * Greiner, N. et al. Recruitment of upper-limb motoneurons with epidural
electrical stimulation of the primate cervical spinal cord. _Nat. Commun._ 12, 1–19 (2021). Article CAS Google Scholar * Barra, B. et al. A versatile robotic platform for the design of
natural, three-dimensional reaching and grasping tasks in monkeys. _J. Neural Eng_. https://doi.org/10.1088/1741-2552/ab4c77 (2019). * Capogrosso, M. et al. A brain–spine interface
alleviating gait deficits after spinal cord injury in primates. _Nature_ 539, 284–288 (2016). Article PubMed PubMed Central Google Scholar * Schiavone, G. et al. Soft, implantable
bioelectronic interfaces for translational research. _Adv. Mater._ 32, 1906512 (2020). Article CAS Google Scholar * Chao, Z. C., Sawada, M., Isa, T. & Nishimura, Y. Dynamic
reorganization of motor networks during recovery from partial spinal cord injury in monkeys. _Cereb. Cortex_ https://doi.org/10.1093/cercor/bhy172 (2018). * Freund, P. et al. Nogo-A–specific
antibody treatment enhances sprouting and functional recovery after cervical lesion in adult primates. _Nat. Med._ 12, 790–792 (2006). Article CAS PubMed Google Scholar * Sharpe, A. N.
& Jackson, A. Upper-limb muscle responses to epidural, subdural and intraspinal stimulation of the cervical spinal cord. _J. Neural Eng._ 11, 016005 (2014). Article PubMed PubMed
Central Google Scholar * Gallego, J. A., Perich, M. G., Miller, L. E. & Solla, S. A. Neural manifolds for the control of movement. _Neuron_ 94, 978–984 (2017). Article CAS PubMed
PubMed Central Google Scholar * Kato, K., Nishihara, Y. & Nishimura, Y. Stimulus outputs induced by subdural electrodes on the cervical spinal cord in monkeys. _J. Neural Eng._ 17,
016044 (2020). Article PubMed Google Scholar * de Freitas, R. M. et al. Selectivity and excitability of upper-limb muscle activation during cervical transcutaneous spinal cord stimulation
in humans. _J. Appl. Physiol._ 131, 746–759 (2021). Article CAS PubMed Google Scholar * Kirsch, R. & Rymer, W. Neural compensation for muscular fatigue: evidence for significant
force regulation in man. _J. Neurophysiol._ 57, 1893–1910 (1987). Article CAS PubMed Google Scholar * Song, S. & Geyer, H. A neural circuitry that emphasizes spinal feedback
generates diverse behaviours of human locomotion. _J. Physiol._ https://doi.org/10.1113/JP270228 (2015). Article PubMed PubMed Central Google Scholar * Seáñez, I. & Capogrosso, M.
Motor improvements enabled by spinal cord stimulation combined with physical training after spinal cord injury: review of experimental evidence in animals and humans. _Bioelectron. Med._ 7,
1–13 (2021). Article Google Scholar * Granat, M., Heller, B., Nicol, D., Baxendale, R. & Andrews, B. Improving limb flexion in FES gait using the flexion withdrawal response for the
spinal cord injured person. _J. Biomed. Eng._ 15, 51–56 (1993). Article CAS PubMed Google Scholar * Jenny, A. B. & Inukai, J. Principles of motor organization of the monkey cervical
spinal cord. _J. Neurosci._ 3, 567–575 (1983). Article CAS PubMed PubMed Central Google Scholar * National Research Council (US) Institute for Laboratory Animal Research. _Guide for the
Care and Use of Laboratory Animals_ (National Academies Press (US), 1996). * Mathis, A. et al. DeepLabCut: markerless pose estimation of user-defined body parts with deep learning. _Nat.
Neurosci._ 21, 1281–1289 (2018). Article CAS PubMed Google Scholar * Toossi, A. et al. Effect of anesthesia on motor responses evoked by spinal neural prostheses during intraoperative
procedures. _J. Neural Eng._ 16, 036003 (2019). Article PubMed Google Scholar * Teulings, H.-L., Contreras-Vidal, J. L., Stelmach, G. E. & Adler, C. H. Parkinsonism reduces
coordination of fingers, wrist, and arm in fine motor control. _Exp. Neurol._ 146, 159–170 (1997). Article CAS PubMed Google Scholar * Gallego, J. A., Perich, M. G., Chowdhury, R. H.,
Solla, S. A. & Miller, L. E. Long-term stability of cortical population dynamics underlying consistent behavior. _Nat. Neurosci._ 23, 260–270 (2020). Article CAS PubMed PubMed Central
Google Scholar * Raspopovic, S., Capogrosso, M. & Micera, S. A computational model for the stimulation of rat sciatic nerve using a transverse intrafascicular multichannel electrode.
_IEEE Trans. Neural Syst. Rehabil. Eng._ 19, 333–344 (2011). Article PubMed Google Scholar Download references ACKNOWLEDGEMENTS We thank J. Maillard and L. Bossy for the care provided to
the animals; E. Schmidlin and S. Borgognon for their help with anesthesia and surgery preparations; M. Badi for her help and advice during experiment preparations and experimental
procedures; A. Zbinden for her contribution to the health survey of the monkeys; A. Gaillard and A. Francovich for their help with the implementation of the hardware; and students of the
University of Fribourg A. Jeanneret, A. Jelusic, L. M. Jacquemet and S. Borra for their help in processing data. We acknowledge the financial support from the Wyss Center grant (no. WCP 008)
to M.C., G.C. and T.M.; an industrial grant from GTX Medicals to G.C. and M.C; the Bertarelli Foundation (Catalyst Fund Grant to M.C. and T.M. and funds to S.L.); a Swiss National Science
Foundation Ambizione Fellowship (no. 167912 to M.C.) and a Swiss National Science Foundation Doc-Mobility Grant (no. 188027 to B.B.); the European Union’s Horizon 2020 research and
innovation program under the Marie Skłodowska-Curie grant agreement no. 665667 (G.S.); the Swiss National Foundation grant no. BSCGI0_157800 (S.L.); a Whitaker International Scholars Program
fellowship to M.G.P.; and an internal pilot grant of the University of Fribourg to M.C. AUTHOR INFORMATION Author notes * These authors contributed equally: Beatrice Barra, Sara Conti.
AUTHORS AND AFFILIATIONS * Platform of Translational Neuroscience, Department of Neuroscience and Movement Sciences, Faculty of Sciences and Medicine, University of Fribourg, Fribourg,
Switzerland Beatrice Barra, Sara Conti, Katie Zhuang, Maude Delacombaz, Mélanie Kaeser, Eric M. Rouiller & Marco Capogrosso * Rehab and Neural Engineering Labs, University of Pittsburgh,
Pittsburgh, PA, USA Beatrice Barra & Marco Capogrosso * Department of Fundamental Neuroscience, Faculty of Medicine, University of Geneva, Geneva, Switzerland Matthew G. Perich &
Tomislav Milekovic * Bertarelli Foundation Chair in Neuroprosthetic Technology, Laboratory for Soft Bioelectronic Interfaces, Institute of Microengineering, Institute of Bioengineering,
Centre for Neuroprosthetics, École Polytechnique Fédérale de Lausanne, Geneva, Switzerland Giuseppe Schiavone, Florian Fallegger & Stephanie Lacour * Center for Neuroprosthetics and
Brain Mind Institute, School of Life Sciences, École Polytechnique Fédérale de Lausanne, Lausanne, Switzerland Katia Galan, Nicholas D. James, Quentin Barraud & Grégoire Courtine *
Defitech Center for Interventional Neurotherapies (NeuroRestore), University Hospital Lausanne (CHUV), University of Lausanne (UNIL) and École Polytechnique Fédérale de Lausanne, Lausanne,
Switzerland Katia Galan, Quentin Barraud, Maude Delacombaz, Tomislav Milekovic, Jocelyne Bloch & Grégoire Courtine * Department of Neurosurgery, CHUV, Lausanne, Switzerland Jocelyne
Bloch & Grégoire Courtine * Department of Neurological Surgery, University of Pittsburgh, Pittsburgh, PA, USA Marco Capogrosso Authors * Beatrice Barra View author publications You can
also search for this author inPubMed Google Scholar * Sara Conti View author publications You can also search for this author inPubMed Google Scholar * Matthew G. Perich View author
publications You can also search for this author inPubMed Google Scholar * Katie Zhuang View author publications You can also search for this author inPubMed Google Scholar * Giuseppe
Schiavone View author publications You can also search for this author inPubMed Google Scholar * Florian Fallegger View author publications You can also search for this author inPubMed
Google Scholar * Katia Galan View author publications You can also search for this author inPubMed Google Scholar * Nicholas D. James View author publications You can also search for this
author inPubMed Google Scholar * Quentin Barraud View author publications You can also search for this author inPubMed Google Scholar * Maude Delacombaz View author publications You can also
search for this author inPubMed Google Scholar * Mélanie Kaeser View author publications You can also search for this author inPubMed Google Scholar * Eric M. Rouiller View author
publications You can also search for this author inPubMed Google Scholar * Tomislav Milekovic View author publications You can also search for this author inPubMed Google Scholar * Stephanie
Lacour View author publications You can also search for this author inPubMed Google Scholar * Jocelyne Bloch View author publications You can also search for this author inPubMed Google
Scholar * Grégoire Courtine View author publications You can also search for this author inPubMed Google Scholar * Marco Capogrosso View author publications You can also search for this
author inPubMed Google Scholar CONTRIBUTIONS M.C., B.B. and S.C. conceived the study. B.B., M.G.P. and T.M. designed and implemented the hardware and software tools. S.C. designed the
behavioral task and training strategy. G.S., F.F. and S.L. designed and manufactured the implantable interface. B.B., S.C., M.G.P. and M.C. conducted the experiments. B.B., S.C., M.G.P. and
K.Z. performed the data analysis. S.C., M.D. and M.K. trained the animals. S.C., K.G., N.D.J. and Q.B. processed the histological data. J.B., G.C. and M.C. designed surgical implantation
strategies and stimulation strategies. G.C. and J.B. performed surgical implantations and lesions. E.M.R. and M.C. implemented and supervised procedures on monkeys. M.C., B.B., S.C. and
M.G.P. wrote the manuscript. All authors edited the manuscript. S.L., T.M., J.B., G.C. and M.C. secured funding for the study. M.C. supervised the study. CORRESPONDING AUTHOR Correspondence
to Marco Capogrosso. ETHICS DECLARATIONS COMPETING INTERESTS G.C., J.B., S.L., M.C., B.B. and K.Z. hold various patents in relation to the present work. G.C., S.L. and J.B. are founders and
shareholders of Onwarrd Medical, a company developing an EES-based therapy to restore movement after spinal cord injury. M.C. is a founder and shareholder of Reach Neuro, Inc., a company
developing spinal cord stimulation technologies for stroke. All other authors declare no competing interests. PEER REVIEW PEER REVIEW INFORMATION _Nature Neuroscience_ thanks Sliman
Bensmaia, Andrew Jackson and Arthur Prochazka for their contribution to the peer review of this work. ADDITIONAL INFORMATION PUBLISHER’S NOTE Springer Nature remains neutral with regard to
jurisdictional claims in published maps and institutional affiliations. EXTENDED DATA EXTENDED DATA FIG. 1 MONKEY’S SPECIFIC MOVEMENT PERFORMANCES. (A) Portfolio of signals recorded during
intact movement for each animal. These signals have been recorded during the experimental session prior to the lesion. Motor cortex recordings show firing rate profiles for the 64
microelectrodes. Each row shows the firing rate of a specific electrode. Electrodes are displayed from top to bottom by order of first activation in a reference trial. Arbitrary units in
motor cortex recording indicate normalized firing rate for each electrode (see Methods). In kinematic and EMG plots, black lines correspond to the mean profile across all trials, shaded area
shows the SEM across all trials. Kinematic scales are expressed in mm. For Mk-Yg, arbitrary units on kinematic plots represent displacement units derived by the count of video pixels. EMG
scales are expressed in mV. (B) Kinematic strategies implemented by each monkey. Stick diagrams representations of the arm kinematic during reach (blue) and pull (yellow). The black line
highlights the elbow trajectory. Pie charts represent the percentage of success and failure in task performance before lesion. (C) Offline decoding performance for Mk-Br and Mk-Yg before
lesion. Histograms show timing accuracy of reach (blue) and pull (yellow) event decoding. The height of bars (y coordinate) illustrates the amount of events decoded with a specific timing
accuracy (x coordinate). Pie charts (inset) show the percentage of correctly identified (true positive) reaches (blue) and pulls (yellow), across all decoded events. The black portion of the
pie chart highlights the percentage of false positive decoded events. EXTENDED DATA FIG. 2 ELECTRODE ARRAY PERSONALIZATION. (A) Anatomical landmarks used to tailor the epidural interface to
each monkey’s anatomy (Length of dorsal aspect of spinal canal Lcs, length of C5-T1 spinal segment LC5-T1, electrode width Wel, electrode length Lel). Three-dimensional reconstructions of
vertebras are obtained by CT-reconstruction (Osirix, Pixmeo, Switzerland). (B) Personalized design of the epidural implant for each animal. All measures are in millimeters. Yellow traces at
the bottom of the electrode identify connectors. (C) Position stability of the epidural array over time, illustrated through X-rays imaging taken during 3 consecutive weeks after the
implantation, images from Mk-Yg (D) Compression injury at the insertion level of the array (T2-T3 segment) in Mk-Br, discovered post-mortem, stained with NeuN (neuronal cell bodies) and Iba1
(microglia). EXTENDED DATA FIG. 3 RECRUITMENT CURVES. Muscle recruitment obtained by stimulating, through different electrode contacts (E1, E2, E3, E5), at 1 Hz at C5, C6/C7, and T1 spinal
segments for Mk-Br and Mk-Sa. Mk-Sa only had three muscles implanted: biceps, triceps, and flexor digitorium superficialis. EXTENDED DATA FIG. 4 GRADED MUSCLE ACTIVATION DURING TRAIN PULSES.
(A) Energy of EMG signals of triceps (Mk-Br and Mk-Yg), Flexor Digitorium Superficialis (Mk-Yg) and abductor pollicis (Mk-Br) muscles, following pulse-train stimulation at different
frequencies (on the x-axis). Black bullets represent mean values. (B) Evolution over time of the peak-to-peak value of stimulation evoked responses during a stimulation burst. Each plot
shows the evolution for a specific muscle following pulse-train stimulation at 50 and 100 Hz. Triceps is shown for Mk-Br and Mk-Yg, Flexor Digitorium Superficialis for Mk-Yg and abductor
pollicis for Mk-Br. Each data point is represented as a bullet and lines represent mean values over time. EXTENDED DATA FIG. 5 DESIGN OF STIMULATION PROTOCOL. (A) Combined representation of
movement smoothness, elbow and finger flexion, and pulling force during anesthetized stimulation. Shades of gray highlight three frequency ranges that produce: (1) smooth trajectory, but
little movement and low force (20 Hz), (2) smooth trajectory, extended movement and medium force (40 and 50 Hz), (3) abrupt and very extended movement and low force (80 and 100 Hz).
Kinematics and force reported here were measured in different experiments, kinematics was unconstrained, force data were acquired in isometric conditions (see Methods). The range 40-50 Hz
was selected as the best optimization of sufficient movement, smoothness and force production. (B) Schematic representation of arm and hand kinematics during stimulation delivered from the
selection of three contacts to produce elbow extension (blue), hand and wrist flexion (yellow and black), and elbow flexion (yellow). (C) Example of comparison between EMG activity during
intact movement (left) and movement elicited by chaining stimulation from the three selected contacts (right). (D) Scheme illustrating how stimulation is triggered from movement-related
intra-cortical signals. On the right, online performances of movement attempt decoder in two animals with SCI. Pie charts represent percentage of predicted (blue) and unpredicted (black)
reach events by our decoder. EXTENDED DATA FIG. 6 KINEMATIC IS MODULATED BY STIMULATION FREQUENCY. (A) Stick diagram schematic of movements elicited by pulse-trains of stimulation in
anesthetized conditions. Mk-Br: on the left, arm kinematic obtained by delivering stimulation at different frequencies from contact number 5, on the bottom-left, arm kinematics obtained by
repetitive delivery of a burst at 50 Hz; on the bottom right, superimposition of stick diagrams obtained with stimulation at 20 Hz and at higher frequencies (50 or 100 Hz) from different
contacts. For Mk-Yg: arm kinematic obtained by delivering stimulation at different frequencies from contact number 2 and superimposition of stick diagrams obtained with stimulation at 20 Hz
and at higher frequencies (50 or 100 Hz) from different contacts. (B) On the left, finger flexion produced by stimulation at different frequencies from the grasp contact in Mk-Br. Black
bullets represent the mean value across different pulse-trains. On the right, wrist flexion obtained by stimulation at different frequencies from the grasp contact in Mk-Yg. EXTENDED DATA
FIG. 7 PERFORMANCE EVOLUTION. (A) Evolution (in weeks) of rates at which Mk-Br performed reach movements after SCI (black), compared to the performances before injury (gray). (B) Evolution
(in weeks) of rates at which Mk-Br performed grasp movements after SCI (black), compared to the performances before injury (gray). (C) Evolution (in weeks) of rates at which Mk-Br performed
pull movements after SCI (black), compared to the performances before injury (gray). (D) Evolution (in days) of pull force after SCI without stimulation for Mk-Br. Values are plotted as the
mean ± STD (from left to right, n = 28, 29, 22, 26, 51 independent samples). Statistical analysis was carried out with two-sided Wilcoxon Ranksum test and Tuckey-Cramer correction. (E)
Evolution (in weeks) of rates at which Mk-Yg performed reach movements after SCI (black), compared to the performances before injury (gray). (F) Evolution (in weeks) of rates at which Mk-Yg
performed grasp movements after SCI (black), compared to the performances before injury (gray). (G) Evolution (in weeks) of rates at which Mk-Yg performed pull movements after SCI (black),
compared to the performances before injury (gray). (H) Evolution (in days) of pull force after SCI without stimulation for Mk-Yg. Values are plotted as the mean ± STD. (from left to right, n
= 35, 23, 14, 20, independent samples). Statistical analysis was carried out with two-sided Wilcoxon Ranksum test and Tuckey-Cramer correction. Source data EXTENDED DATA FIG. 8 EFFECT OF
STIMULATION DURATION AND TIMING. (A) Bar plots report the rate of successful movements after SCI, without stimulation (black), with continuous stimulation (gray) and with phase-dependent
stimulation (blue or yellow) for Mk-Br and Mk-Yg. Data are presented as mean ± STD and normalized on the mean value in stimulation condition. Significance evaluated by estimating two side
residuals via Bootstrap. (B) Left: wrist frontal displacement in trials in which pull stimulation was erroneously triggered during reach (gray and yellow), compared to trials in which pull
stimulation was not delivered (black, solid line represents the mean and shaded area represents the SEM). Yellow bullets highlight the instant at which stimulation was delivered: yellow
lines highlight the trajectories during and after stimulation. Middle: barplot of the length of the reach movement when pull stimulation was erroneously delivered (n = 4) and when pull
stimulation was not delivered (n = 9). Data are presented as mean ± STD. Statistics performed with two-sided Wilcoxon Ranksum test. Right: stick diagram of arm kinematics during reach
without (black) and with (yellow) erroneous pull stimulation. Source data SUPPLEMENTARY INFORMATION SUPPLEMENTARY INFORMATION The file contains supplementary text, data, figures and video
captions. REPORTING SUMMARY SUPPLEMENTARY VIDEO 1 Single-joint movements elicited by pulse-trains of EES at different segmental locations. Shoulder abduction: stimulation at C5; elbow
extension: stimulation at C7; finger flexion: stimulation at T1; reach, grasp and pull sequence: cascade stimulation at C7, T1 and C5. SUPPLEMENTARY VIDEO 2 Effects of EES on reach movement
performance on Mk-Sa. Top left, lateral vision of the animal performing the task; bottom left, delivered stimulation pulses; top right, electromyographic activity from deltoid, biceps and
triceps muscles; bottom right, neural activity from M1 and PMd cortex. SUPPLEMENTARY VIDEO 3 Effects of brain-controlled EES on reach and pull movement performance on Mk-Br. Top left,
lateral vision of the animal performing the task; middle left, delivered stimulation pulses; bottom left, pulling force applied on the robot end effector; top right, neural activity from S1,
M1 and PMd cortex; bottom right, electromyographic activity from deltoid, flexor carpi radialis and abductor pollicis. SUPPLEMENTARY VIDEO 4 Effects of EES on pull movement performance on
Mk-Yg. Top left, lateral vision of the animal performing the task; middle left, delivered stimulation pulses; bottom left, pulling force applied on the robot end effector; top right, neural
activity from S1, M1 and PMd cortex; bottom right, electromyographic activity from biceps, triceps, extensor digitorium communis and flexor digitorium superficialis. SUPPLEMENTARY VIDEO 5
Effects of an EES burst optimized to recover pull, delivered during a reach movement on Mk-Yg. Lateral view of the animal performing the task. SOURCE DATA SOURCE DATA FIG. 2 Statistical
source data. SOURCE DATA FIG. 3 Statistical source data. SOURCE DATA FIG. 4 Statistical source data. SOURCE DATA FIG. 5 Statistical source data. SOURCE DATA FIG. 6 Statistical source data.
SOURCE DATA EXTENDED DATA FIG. 7 Statistical source data. SOURCE DATA EXTENDED DATA FIG. 8 Statistical source data. RIGHTS AND PERMISSIONS Reprints and permissions ABOUT THIS ARTICLE CITE
THIS ARTICLE Barra, B., Conti, S., Perich, M.G. _et al._ Epidural electrical stimulation of the cervical dorsal roots restores voluntary upper limb control in paralyzed monkeys. _Nat
Neurosci_ 25, 924–934 (2022). https://doi.org/10.1038/s41593-022-01106-5 Download citation * Received: 05 April 2021 * Accepted: 19 May 2022 * Published: 30 June 2022 * Issue Date: July 2022
* DOI: https://doi.org/10.1038/s41593-022-01106-5 SHARE THIS ARTICLE Anyone you share the following link with will be able to read this content: Get shareable link Sorry, a shareable link
is not currently available for this article. Copy to clipboard Provided by the Springer Nature SharedIt content-sharing initiative