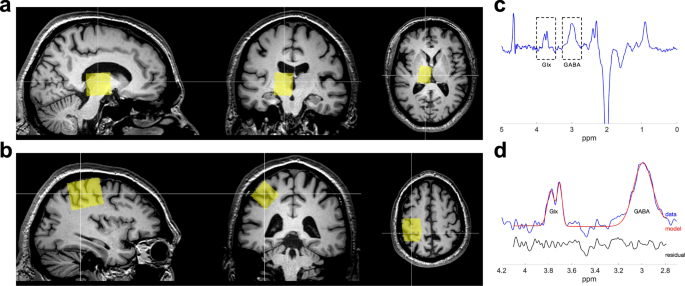
- Select a language for the TTS:
- UK English Female
- UK English Male
- US English Female
- US English Male
- Australian Female
- Australian Male
- Language selected: (auto detect) - EN
Play all audios:
ABSTRACT Impulsivity is inherent to behavioral disorders such as substance abuse and binge eating. While the role of dopamine in impulse behavior is well established, γ-aminobutyric acid
(GABA) therapies have promise for the treatment of maladaptive behaviors. In Parkinson disease (PD), dopaminergic therapies can result in the development of impulsive and compulsive
behaviors, and this clinical syndrome shares similar pathophysiology to that seen in addiction, substance abuse, and binge-eating disorders. We hypothesized that impulsive PD patients have a
reduced thalamic GABAergic response to dopamine therapy. To test this hypothesis, we employed GABA magnetic resonance spectroscopy, D2-like receptor PET imaging, and clinical and
quantitative measures of impulsivity in PD patients (_n_ = 33), before and after dopamine agonist administration. We find a blunted thalamic GABA response to dopamine agonists in patients
with elevated impulsivity (_p_ = 0.027). These results emphasize how dopamine treatment differentially augments thalamic GABA concentrations, which may modify behavioral impulsivity. SIMILAR
CONTENT BEING VIEWED BY OTHERS IMPAIRED STRIATAL GLUTAMATE/GABA REGULATION IN VIOLENT OFFENDERS WITH ANTISOCIAL PERSONALITY DISORDER AND PSYCHOPATHY Article Open access 07 February 2024
PRAMIPEXOLE RESTORES BEHAVIORAL INHIBITION IN HIGHLY IMPULSIVE RATS THROUGH A PARADOXICAL MODULATION OF FRONTOSTRIATAL NETWORKS Article Open access 09 February 2024 CHARACTERIZATION OF BASAL
GANGLIA VOLUME CHANGES IN THE CONTEXT OF HIV AND POLYSUBSTANCE USE Article Open access 14 March 2022 INTRODUCTION Therapies that modulate γ-aminobutyric acid (GABA) have been assessed in
addiction and mood disorders, and are a promising treatment option1, especially for use in alcohol dependence2 substance abuse, and bipolar disorder. Commonly studied medications include
baclofen, a GABAB agonist, and anti-epileptic treatments (e.g., topiramate)3. While the localization for where these treatments exert their effect is unknown, it is well-appreciated that
altered mesolimbic and mesocortical networks subserve maladaptive behaviors. Impulsivity is often discussed in the context of the cognitive process that underlies these behavioral
manifestations4,5,6,7, and is closely linked to reward-based decision making. Reward responsivity is modulated by the mesolimbic dopamine pathway, especially dopamine release in the ventral
striatum. Understanding how this increase can be augmented through interventions that target GABA requires advancements in methods that can assess the neurobiology of GABA, dopamine,
impulsivity, and human behavior. In Parkinson disease (PD), 15–40% of patients treated with dopamine medications can develop maladaptive behaviors8,9 that are characterized by compulsive
participation in naturally occurring rewards, commonly referred to as impulsive and compulsive behaviors (ICB)10,11. This clinical syndrome is of important translational relevance to the
study of dopamine and reward-based decision making9,12, as behaviors emerge as a result of dopamine treatments for motor symptoms13,14,15. PD patients with ICBs compulsively engage in
reward-based activities that frequently include increased time spent on hobbies, compulsive eating, gambling, spending, and/or hypersexuality16,17,18. Dopamine agonists (DAA) therapies
induce greater risk-taking in ICB patients19,20 and while the prevalence is uncommon with L-DOPA monotherapy18,21, when treatment doses are reduced, or discontinued, patients often note the
resolution of the behavioral symptom. In this context, the study of ICBs can provide important advances in allowing for translational studies that assess GABAergic neurotransmitter response
to dopamine treatments, and their relevance to behavioral symptoms in humans. DAA preferentially target D3 and D2 receptors (D2-like receptors), with less affinity to D122. This class of
medications is one of the strongest risk factors for developing ICBs, not only in PD but also in other disorders such as restless leg syndrome23. The nature of this medication’s side
effects, the use of these medications in routine practice, and willingness for patients to withhold medications over an extended period of time, provide a unique opportunity to devise
studies that assess neurobiological effects of medication use. These studies have expanded our understanding that DAA augments metabolic activity in ventral striatum circuitry24, and modify
reward-based circuits that connect mesolimbic and mesocortical regions25,26. The effect of dopamine therapies on thalamic function and associated networks has not been a consistent focus of
research. Thalamocortical networks are largely modulated by excitatory/inhibitory outputs arising from the globus pallidus and subthalamic nucleus, but emerging data suggest dopamine itself
may regulate this network through concerted action on thalamic-based dopamine receptors27,28. The main neurochemical regulator of thalamocortical inhibition is GABA, and several studies
point to thalamic GABA dysfunction in PD29,30,31. We hypothesized that patients with ICB will demonstrate a blunted thalamic GABAergic response to DAA treatment and a net-excitatory effect
from direct-pathway stimulation on the thalamocortical network. In this study, a within-patient design allowed us to assess the effect of DAA medication on thalamic GABA in PD patients
manifesting with ICBs, and determine how thalamic D2-like receptors modulate this effect. For this purpose, we employed J-edited magnetic resonance spectroscopy (MRS), which enables
simultaneous quantification of GABA and glutamate (as Glx, a complex of glutamate and glutamine) in humans non-invasively in vivo. Finally, we conducted an exploratory analysis to evaluate
if the medication effect on thalamic GABA was mediated by thalamic D2-like dopamine receptor availability, as measured by PET imaging with [18F]-Fallypride, a high-affinity D2-like receptor
ligand that can measure D2/3 non-displaceable binding potential (BPND). RESULTS PARTICIPANTS All participants provided informed, written consent for this prospective study. This study
enrolled 33 participants with PD (19 males, median age = 64 years; range = 45–80 years), who underwent neuroimaging, cognitive, and neurological assessments before and after DAA
administration. Nineteen patients had a clinical diagnosis of active ICB symptoms (ICB+), based on clinical evaluation. The predominant behavior was compulsive eating in 16 participants,
with the other 3 participants noting hypersexual behavior in addition to compulsive eating. Demographic and clinical features are presented in Table 1. All participants completed two MRI
sessions: following withdrawal from their dopaminergic medication (Off-DAA), and in their optimal state of DAA therapy (On-DAA). GABAERGIC RESPONSE TO DOPAMINE AGONIST J-edited MRS was used
to non-invasively quantify GABA and glutamate concentration separately in the thalamus and motor cortex before and after DAA medication administration. Figure 1 shows voxel placement and a
representative spectrum. Thalamic GABAergic response to DAA (estimated as ΔGABA = [GABAON – GABAOFF]/GABAOFF, where GABAON,OFF represent the thalamic GABA+ estimate in the On- and Off-DAA
conditions) was significantly blunted for ICB+ vs. ICB− (_p_ = 0.045) while accounting for age and DAA dosage, but this difference was not significant for the motor cortex GABA (_p_ =
0.486). Moreover, thalamic ΔGABA was significantly correlated to self-reported ratings of impulsivity as defined by the Questionnaire for Impulsive-Compulsive Disorders in Parkinson’s
Disease-Rating Scale (QUIP-RS). Here, lower thalamic changes in GABA correlated with greater impulsivity (adjusted for age and DAA dosage) (Fig. 2) (_p_ = 0.027). No relationship was
observed between ΔGABA in the motor cortex and impulsivity (_p_ = 0.216). The thalamic glutamatergic response (measured using the Glx levels) to DAA was not significantly different between
ICB+ and ICB− (_p_ = 0.305), and it was not associated with QUIP scores (_p_ = 0.389). ASSOCIATION BETWEEN THALAMIC GABAERGIC RESPONSE TO DOPAMINE AGONIST AND DOPAMINE RECEPTOR AVAILABILITY
Figure 3 shows an example of the [18F]fallypride BPND values in the thalamus. Across all participants, we did not observe an association between thalamic ΔGABA and D2-like receptor
availability, while adjusting for age (_p_ = 0.973). However, we observed that this relationship appears to differ between ICB+ and ICB− patients (_p_ = 0.051) (Fig. 4). DISCUSSION
Interrogating the effect of dopamine on the direct and indirect pathway in humans has generally been difficult, owing to a lack of ability to measure GABA concentration, limitations in
isolating and interpreting network-based activation of dopamine receptor subtypes, and inability to understand concerted effects of these manipulations on human behavior. Neurotransmitter
investigations are frequently conducted under pharmacological manipulation in sedated animals32,33, or with tissue biopsy or autopsy procedures, with the obvious caveat that in vitro
chemical profiles may differ from their in vivo counterparts34, and anesthesia may confound neurotransmitter measurements. This study is an important translational extension of decades of
research assessing dopamine effects on reward and behavior, as we are able to measure the effect of dopamine receptor agonists on in vivo thalamic GABA concentrations in humans using
MRS35,36,37. Our results support a clear link between patient-reported assessments of reward responsiveness, the clinical-defined severity of compulsive reward-driven behaviors in the
determination of ICB (compulsive eating and hypersexuality, emerging in time after the initiation therapy for motor symptoms of PD), and thalamic GABA response to DAA. If the effect of GABA
at the thalamic level is indeed linked to behavioral symptoms of compulsive reward-based behaviors, future treatments that can focus on localized modification of GABA, based on clinical and
radiologic data, may allow for improved target validation, patient identification, and therapeutic effect. Dopamine modulates basal ganglia circuitry through the direct and indirect
pathways, and while conventional models reference the motor effects of these pathways, new models incorporate effects on decision making and behavior38. In rodents, direct pathway
stimulation results in compulsive reward-driven behavior, and indirect pathway stimulation results in more flexible decision making39. Common to both pathways is thalamocortical circuitry,
where stimulation of the direct pathway (D1 receptors) results in reduced thalamic inhibition of excitatory thalamocortical signaling, while stimulation of the indirect pathway (D2-like
receptors) results in inhibition of this pathway40,41. Dopamine signaling plays an important role in behavioral regulation and decision making42,43,44, and dopamine-induced changes to the
balance of the direct and indirect activation may provide a biological basis for medication effects that manifest with impulsive and compulsive reward-based behaviors. Previous studies of
compulsive eating and hypersexuality have linked thalamic signaling to behavioral manifestations of appetitive motivation. For instance, differential GABA agonism in the paraventricular
nucleus or medial dorsal nucleus of the thalamus can increase or suppress appetite45. Likewise, thalamic activation in response to sexual stimuli is greater in patients with hypersexual
behavior46. We interpret the difference in DAA-induced thalamic GABA to emphasize a biological effect of this medication effect on the balance of the direct and indirect pathway activation.
These pathways operate in a concert given their opposing effect on movement and behavior. The relative absence of a thalamic GABA response in patients with ICB suggests a greater direct
pathway stimulation or blunted indirect pathway activation. Previous studies that employ optogenetic techniques in assessments of rodent behavior indicate that activation of direct pathway
neurons in the ventral striatum (i.e., nucleus accumbens) induce a behavior similar to what is encountered in patients with ICBs39. These compulsive or persistent pursuits are of naturally
rewarding behaviors (e.g., shopping, gambling, eating, and sex). We cannot rule out the possibility that the medication effect acts on thalamic dopamine receptors. We have previously
described reduced D2-like receptors in the thalamus of PD patients47, but have not seen differences in patients with and without ICBs48. D2-like receptors in the thalamus are highly
concentrated in the medial dorsal nucleus, and anterior nucleus49 (Fig. 3). As only a subset of the participants in the study (_n_ = 20) underwent the PET scans, these results should be
interpreted carefully with the expectation that a larger sample size may capture relationships not currently evident. These nuclei are important in modulating decision making and behavior,
thus we speculate that the GABAergic response is localized here, hence the relation to behavioral symptoms in patients. The limitation of MRS is that we cannot provide accurate localization
to specific nuclei of the thalamus. Furthermore, the lack of change in motor cortex GABA50, suggests that the medication effect may be localized subcortically, where future studies should
assess striatal GABA changes in response to dopamine therapies. In summary, these data provide insights into the effect of dopamine therapy on non-dopamine-related neurotransmission, namely
GABA concentration. The findings of altered response based on clinical presentation and patient-reported outcomes measure are important translational efforts linking dopamine effects on
reward, basal ganglia, and thalamocortical circuitry. These data have potential relevance not only to PD but also to studies of addiction science. METHODS PARTICIPANTS Patients with
idiopathic PD meeting UK Brain Bank criteria51 treated with DAA therapy were recruited from the Movement Disorders Clinic at Vanderbilt University Medical Center. The study has been carried
out in accordance with The Declaration of Helsinki, and all subjects provided written, informed consent before participating in the study in compliance with the standards of ethical conduct
in human investigation regulated by the Vanderbilt Institutional Review Board. PD severity was assessed by a board-certified neurologist using the Movement Disorders Society-Unified
Parkinson’s Disease Rating Scale (MDS-UPDRS) parts II and III. These scales assess the self-reported quality of life and motor symptom severity, respectively52. The cognitive screening was
performed using the Montreal Cognitive Assessment (MoCA) to exclude patients with frank dementia53, requiring a score of at least 22, and depression symptoms were screened using the Center
for Epidemiologic Studies Depression Scale Revised (CESD-R)54. Patients’ current prescribed dosages of dopaminergic medication, including Levodopa and DAA, were converted to levodopa
equivalent daily dose (LEDD) using the conversion factors and formulae reported in ref. 55. Patients were excluded if they had (i) history of neurological diseases other than PD, (ii)
clinical symptoms of dementia, depression, cerebrovascular, or cardiovascular disease, (iii) an implanted deep brain stimulator, or (iv) implanted hardware that was contraindicated for 3 T
magnetic resonance imaging (MRI). Patients were categorized as current (or active) ICB+ or ICB− based on a detailed semi-structured behavioral interview with the patient and spouse. This
interview evaluated the presence of compulsive behaviors with onset following DAA administration, with specific attention towards the previously reported categories of compulsive shopping,
eating, hypersexuality, gambling, and hobbyism56,57,58. Prior to the interview, participants completed two self-report scales: Questionnaire for Impulsive-Compulsive Disorders in Parkinson’s
Disease-Rating Scale (QUIP-RS)58, and Barrett Impulsiveness Scale (BIS-11)59. Patients were designated as ICB+ if the criteria for present ICBs as defined in the Diagnostic and Statistical
Manual of Mental Disorders (DSM-IV-TR)60 had emerged following the initiation of DAA. All patients enrolled in the study completed two MRI sessions. One session was performed following
withdrawal from their dopaminergic medication (Off-DAA), and the other was when patients were in their optimal state of DAA therapy (On-DAA). In the Off-DAA condition, patients refrained
from all dopaminergic medications for a total time of 5-half lives of DAA. Practically, this was at least 36 h for DAA, and 16 h for Levodopa due to differences in pharmacokinetic
properties61,62. This period was deemed sufficient to eliminate DAA effects, while minimizing potential patient discomfort63. In the On-DAA state, patients were evaluated after taking their
prescribed DAA medication, having withheld Levodopa for at least 16 h. Extended-release DAA compounds (taken by 12 patients) were administered 6 h before MR scanning, whereas non-extended
release DAA (taken by 18 patients) were administered 2 h before scanning. No changes in medication dosages or addition or discontinuation of either drug for clinical purposes were made at
any time during study participation. MRS ACQUISITION Patients were scanned in the Off- and On-DAA states using a 3 T MRI scanner (Achieva, Philips Healthcare, the Netherlands) with body coil
transmit and 32-channel head coil reception. Scanning included a 3D structural _T__1_-weighted whole-brain image, (MPRAGE, TR/TE = 8.9/4.6 ms; turbo gradient echo factor = 131; spatial
resolution = 1 × 1 × 1 mm3), and single-voxel J-edited MRS using MEGA-PRESS64 (TR/TE = 3000/68 ms; 320 transients; 2048 data points at a spectra width of 2 kHz). The spectroscopy voxels were
planned off orthogonal reconstructions of the high-resolution _T_1-weighted scan and placed in the right thalamic area (voxel dimensions = 30 × 22 × 28 mm3) (Fig. 1a) and the right motor
cortex (voxel dimensions = 40 × 25 × 25 mm3) (Fig. 1b). Editing pulses (14 ms, 140 Hz bandwidth) were applied at 1.9 ppm and 8 ppm on alternate scans. An unedited MRS scan without water
suppression was also acquired for normalization. GABA concentration in the motor cortex was also analyzed as a control region, as we hypothesize that DAA will have a regional effect in
thalamic GABA but will not affect GABA concentration in the motor cortex. In addition, we investigated the effect of DAA on thalamic excitatory activity by measuring thalamic glutamate
concentration. MRS DATA ANALYSIS MRS analysis was performed using Gannet 3.065. Frequency and phase correction and outlier rejection was applied (Fig. 1c). The single GABA+ peak at 3 ppm
(GABA peak with a contribution from macromolecule signals) was fitted using a Gaussian model (Fig. 1d), and the levels of GABA+ were calculated from the area under the peak. The unsuppressed
water spectrum was processed to obtain the area under the water peak, which was then used to estimate GABA+ concentration relative to water. The levels of glutamate were obtained from the
Glx peak (a complex of glutamate and glutamine) at 3.75 ppm (Fig. 1d), which was fitted to a Gaussian model to obtain the area under the peak, and then normalized by the area under the water
peak. To account for the underlying tissue composition, we applied the α-correction66,67. GannetCoRegister was used to register the MRS voxel to the _T__1_-weighted image, and tissue
segmentation was performed by merging the results obtained from FSL FAST and FSL FIRST (Supplementary Fig. 1) (FSL v5.0.2.1, FMRIB, Oxford, UK). The MRS voxel mask was then applied to the
tissue segmentation to determine the tissue voxel fractions for gray matter (GM), white matter (WM), and cerebrospinal fluid (CSF) (Supplementary Fig. 1). The compartment correction (using
_α_ = 0.5, Wanasapura values for relaxation parameters, and 36.1, 43.3, and 53.8 mol/dm3 for MR-visible water concentrations for WM, GM, and CSF, respectively), and tissue normalization were
applied to account for differences in GABA+/water concentration between GM and WM, and to obtain the compartment corrected thalamic and motor cortex GABA+ concentration67. A similar
correction was applied to the Glx/water measures to obtain the compartment corrected Glx concentration. PET ACQUISITION AND PROCESSING A subset of 20 participants (10 ICB+ and 10 ICB−)
completed a PET scan with [18F]Fallypride as described in refs. 47,48. D2-like receptor levels were estimated using the simplified reference tissue model (SRTM) performed in PMOD software
version 3.7 (PMOD Technologies, Zurich Switzerland) to measure [18F]fallypride binding potential (BPND; the ratio of specifically bound [18F]fallypride to its nondisplaceable concentration
as defined under equilibrium conditions)47,48. BPND images were co-registered to the T1-weighted image using FSL FLIRT (FSL v5.0.2.1, FMRIB, Oxford, UK). FSL FIRST was used to obtain the
thalamic mask and the mean BPND values were recorded (Fig. 3). STATISTICAL ANALYSIS The effect of DAAs on thalamic GABA was estimated as ΔGABA = [GABAON – GABAOFF]/GABAOFF, where GABAON,OFF
represent the thalamic GABA+ estimate in the On- and Off-DAA conditions. To understand whether thalamic GABA changes are different between ICB+ and ICB−, we performed a general linear
regression model (GLM) analysis specifying thalamic ΔGABA as dependent variable, ICB status as an independent variable, and age and DAA dosage (i.e., agonist single dose equivalent) as
covariates (GLM: Thalamic ΔGABA ~ ICD status + age + DAA dosage). To evaluate if thalamic GABA changes were related to a quantitative marker of impulsivity, we performed GLM analyses
specifying ΔGABA as the dependent variable, QUIP-RS score as an independent variable, and age and DAA dosage as covariates (GLM: Thalamic ΔGABA ~ QUIP-RS + age + DAA dosage). The above GLM
analyses were also performed for the motor cortex GABA and the thalamic glutamate as dependent variables. Finally, we tested the association between the changes in thalamic GABA and the
thalamic BPND, while adjusting for age (GLM: Thalamic ΔGABA ~ thalamic BPND + age), and we evaluated if this association was different between ICB+ and ICB− patients (GLM: Thalamic ΔGABA ~
thalamic BPND + ICD status + thalamic BPND*ICD status + age). REPORTING SUMMARY Further information on research design is available in the Nature Research Reporting Summary linked to this
article. DATA AVAILABILITY The data presented in this work are available on request from the corresponding author. CODE AVAILABILITY The code used for MRS processing is available on request
from the corresponding author. REFERENCES * Addolorato, G., Leggio, L., Hopf, F. W., Diana, M. & Bonci, A. Novel therapeutic strategies for alcohol and drug addiction: focus on GABA, ion
channels and transcranial magnetic stimulation. _Neuropsychopharmacology_ 37, 163–177 (2012). Article CAS PubMed Google Scholar * Caputo, F. & Bernardi, M. Medications acting on the
GABA system in the treatment of alcoholic patients. _Curr. Pharm. Des._ 16, 2118–2125 (2010). Article CAS PubMed Google Scholar * Addolorato, G. et al. Baclofen in the treatment of
alcohol withdrawal syndrome: a comparative study vs diazepam. _Am. J. Med._ 119, 276.e13 (2006). Article Google Scholar * Bimpisidis, Z. & Wallén-Mackenzie, Å. Neurocircuitry of reward
and addiction: potential impact of dopamine–glutamate co-release as future target in substance use disorder. _J. Clin. Med._ 8, 1887 (2019). Article CAS PubMed Central Google Scholar *
Hammes, J. et al. Dopamine metabolism of the nucleus accumbens and fronto-striatal connectivity modulate impulse control. _Brain_ 142, 733–743 (2019). Article PubMed Google Scholar *
Frank, G. K. W. Neuroimaging and eating disorders. _Curr. Opin. Psychiatry_ 32, 478–483 (2019). Article PubMed PubMed Central Google Scholar * Cavada, C., Compañy, T., Tejedor, J.,
Cruz-Rizzolo, R. J. & Reinoso-Suárez, F. The anatomical connections of the macaque monkey orbitofrontal cortex. A review. _Cereb. Cortex_ 10, 220–242 (2000). Article CAS PubMed Google
Scholar * Garcia-Ruiz, P. J. et al. Impulse control disorder in patients with Parkinson’s disease under dopamine agonist therapy: a multicentre study. _J. Neurol. Neurosurg. Psychiatry_
85, 840–844 (2014). Article PubMed Google Scholar * Weintraub, D., David, A. S., Evans, A. H., Grant, J. E. & Stacy, M. Clinical spectrum of impulse control disorders in Parkinson’s
disease. _Mov. Disord_ 30, 121–127 (2015). Article CAS PubMed Google Scholar * Voon, V. et al. Prevalence of repetitive and reward-seeking behaviors in Parkinson disease. _Neurology_ 67,
1254–1257 (2006). Article CAS PubMed Google Scholar * Bostwick, J. M., Hecksel, K. A., Stevens, S. R., Bower, J. H. & Ahlskog, J. E. Frequency of new-onset pathologic compulsive
gambling or hypersexuality after drug treatment of idiopathic Parkinson disease. _Mayo Clin. Proc._ 84, 310–316 (2009). Article CAS PubMed PubMed Central Google Scholar * Voon, V.,
Potenza, M. N. & Thomsen, T. Medication-related impulse control and repetitive behaviors in Parkinson’s disease. _Curr. Opin. Neurol._ 20, 484–492 (2007). Article PubMed Google Scholar
* Silva, B., Canas-Simião, H. & Cavanna, A. E. Neuropsychiatric aspects of impulse control disorders. _Psychiatr. Clin. North Am._ 43, 249–262 (2020). Article PubMed Google Scholar
* Kelly, M. J., Baig, F., Hu, M. T. M. & Okai, D. Spectrum of impulse control behaviours in Parkinson’s disease: pathophysiology and management. _J. Neurol., Neurosurg. Psychiatry_ 91,
703–711 (2020). Article Google Scholar * Aumann, M. A. et al. Self‐reported rates of impulsivity in Parkinson’s disease. _Ann. Clin. Transl. Neurol._ 7, 437–448 (2020). Article PubMed
PubMed Central Google Scholar * Voon, V. et al. Prevalence of repetitive and reward-seeking behaviors in Parkinson disease. _Neurology_ 67, 1254–1257 (2006). Article CAS PubMed Google
Scholar * Voon, V., Potenza, M. N. & Thomsen, T. Medication-related impulse control and repetitive behaviors in Parkinson’s disease. _Curr. Opin. Neurol._ 20, 484–492 (2007). Article
PubMed Google Scholar * Weintraub, D., David, A. S., Evans, A. H., Grant, J. E. & Stacy, M. Clinical spectrum of impulse control disorders in Parkinson’s disease. _Mov. Disord._ 30,
121–127 (2015). Article CAS PubMed Google Scholar * Voon, V. et al. Dopamine agonists and risk: impulse control disorders in Parkinson’s disease. _Brain_ 134, 1438–1446 (2011). Article
PubMed PubMed Central Google Scholar * Claassen, D. O. et al. The risky business of dopamine agonists in Parkinson disease and impulse control disorders. _Behav. Neurosci._ 125, 492–500
(2011). Article CAS PubMed PubMed Central Google Scholar * Garcia-Ruiz, P. J. et al. Impulse control disorder in patients with Parkinson’s disease under dopamine agonist therapy: a
multicentre study. _J. Neurol. Neurosurg. Psychiatry_ 85, 841–845 (2014). Article Google Scholar * Gerlach, M. et al. Dopamine receptor agonists in current clinical use: comparative
dopamine receptor binding profiles defined in the human striatum. _J. Neural Transm._ 110, 1119–1127 (2003). Article CAS PubMed Google Scholar * Cornelius, J., Tippmann-Peikert, M.,
Slocumb, N., Frerichs, C. & Silber, M. Impulse control disorders with the use of dopaminergic agents in restless legs syndrome: a case-control study. _Sleep_ 33, 81–87 (2010). PubMed
PubMed Central Google Scholar * Claassen, D. O. et al. Mesocorticolimbic hemodynamic response in Parkinson’s disease patients with compulsive behaviors. _Mov. Disord._ 32, 1574–1583
(2017). Article CAS PubMed PubMed Central Google Scholar * Petersen, K. et al. Ventral striatal network connectivity reflects reward learning and behavior in patients with Parkinson’s
disease. _Hum. Brain Mapp._ 39, 509–521 (2018). Article PubMed Google Scholar * Trujillo, P. et al. Dopamine effects on frontal cortical blood flow and motor inhibition in Parkinson’s
disease. _Cortex_ 115, 99–111 (2019). Article PubMed PubMed Central Google Scholar * Arbuthnott, G. W. Thalamostriatal synapses—another substrate for dopamine action? _Brain Res._ 211,
1–11 (2014). Article CAS Google Scholar * Munsch, T., Yanagawa, Y., Obata, K. & Pape, H.-C. Dopaminergic control of local interneuron activity in the thalamus. _Eur. J. Neurosci._ 21,
290–294 (2005). Article PubMed Google Scholar * Dharmadhikari, S. et al. Striatal and thalamic GABA level concentrations play differential roles for the modulation of response selection
processes by proprioceptive information. _Neuroimage_ 120, 36–42 (2015). Article CAS PubMed Google Scholar * Buchanan, R. J. et al. Changes in GABA and glutamate concentrations during
memory tasks in patients with Parkinson’s disease undergoing DBS surgery. _Front. Hum. Neurosci._ 8, 81 (2014). Article PubMed PubMed Central Google Scholar * Ding, S., Li, L. &
Zhou, F. M. Nigral dopamine loss induces a global upregulation of presynaptic dopamine D1 receptor facilitation of the striatonigral GABAergic output. _J. Neurophysiol._ 113, 1697–1711
(2015). Article CAS PubMed Google Scholar * Hyder, F. et al. Neuronal–glial glucose oxidation and glutamatergic–GABAergic function. _J. Cereb. Blood Flow. Metab._ 26, 865–877 (2006).
Article CAS PubMed Google Scholar * Chen, Z., Silva, A. C., Yang, J. & Shen, J. Elevated endogenous GABA level correlates with decreased fMRI signals in the rat brain during acute
inhibition of GABA transaminase. _J. Neurosci. Res._ 79, 383–391 (2005). Article CAS PubMed Google Scholar * Perry, T. L., Hansen, S. & Gandham, S. S. Postmortem changes of amino
compounds in human and rat brain. _J. Neurochem._ 36, 406–412 (1981). Article CAS PubMed Google Scholar * Mescher, M., Merkle, H., Kirsch, J., Garwood, M. & Gruetter, R. Simultaneous
in vivo spectral editing and water suppression. _NMR Biomed._ 11, 266–272 (1998). Article CAS PubMed Google Scholar * Edden, R. A. E. & Barker, P. B. Spatial effects in the
detection of γ-aminobutyric acid: Improved sensitivity at high fields using inner volume saturation. _Magn. Reson. Med._ 58, 1276–1282 (2007). Article CAS PubMed Google Scholar *
Waddell, K. W., Avison, M. J., Joers, J. M. & Gore, J. C. A practical guide to robust detection of GABA in human brain by J-difference spectroscopy at 3 T using a standard volume coil.
_Magn. Reson. Imaging_ 25, 1032–1038 (2007). Article CAS PubMed PubMed Central Google Scholar * Cox, J. & Witten, I. B. Striatal circuits for reward learning and decision-making.
_Nat. Rev. Neurosci._ 20, 482–494 (2019). Article CAS PubMed PubMed Central Google Scholar * Kravitz, A. V., Tye, L. D. & Kreitzer, A. C. Distinct roles for direct and indirect
pathway striatal neurons in reinforcement. _Nat. Neurosci._ 15, 816–818 (2012). Article CAS PubMed PubMed Central Google Scholar * Albin, R. L., Young, A. B. & Penney, J. B. The
functional anatomy of disorders of the basal ganglia. _Trends Neurosci._ 18, 63–64 (1995). Article CAS PubMed Google Scholar * Alexander, G. E., Crutcher, M. D. & Delong, M. R. Basal
ganglia-thalamocortical circuits: parallel substrates for motor, oculomotor, ‘prefrontal’ and ‘limbic’ functions. _Prog. Brain Res._ 85, 119–149 (1990). Article CAS PubMed Google Scholar
* Björklund, A. & Dunnett, S. B. Dopamine neuron systems in the brain: an update. _Trends Neurosci._ 30, 194–202 (2007). Article PubMed Google Scholar * Ikemoto, S., Ikemoto, S.
& Ikemoto, S. Dopamine reward circuitry: two projection systems from the ventral midbrain to the nucleus accumbens-olfactory tubercle complex. _Brain Res. Rev._ 56, 27–78 (2007). Article
CAS PubMed PubMed Central Google Scholar * Volkow, N. D., Fowler, J. S. & Wang, G. J. The addicted human brain: insights from imaging studies. _J. Clin. Investig._ 111, 1444–1451
(2003). Article CAS PubMed PubMed Central Google Scholar * Stratford, T. R. & Wirtshafter, D. Injections of muscimol into the paraventricular thalamic nucleus, but not mediodorsal
thalamic nuclei, induce feeding in rats. _Brain Res._ 1490, 128–133 (2013). Article CAS PubMed Google Scholar * Seok, J. W. & Sohn, J. H. Neural substrates of sexual desire in
individuals with problematic hypersexual behavior. _Front. Behav. Neurosci._ 9, 321 (2015). Article PubMed PubMed Central Google Scholar * Stark, A. J. et al. [18F]fallypride
characterization of striatal and extrastriatal D2/3receptors in Parkinson’s disease. _NeuroImage Clin._ 18, 433–442 (2018). Article PubMed PubMed Central Google Scholar * Stark, A. J. et
al. Nigrostriatal and mesolimbic D2/3 receptor expression in Parkinson’s disease patients with compulsive reward-driven behaviors Nigrostriatal and mesolimbic D 2/3 receptor expression in
Parkinson’s disease. _C. Des. Res. Cite J. Neurosci._ 10, 3082–17 (1523). Google Scholar * Rieck, R. W., Ansari, M. S., Whetsell, W. O., Deutch, A. Y. & Kessler, R. M. Distribution of
dopamine D2-like receptors in the human thalamus: autoradiographic and PET studies. _Neuropsychopharmacology_ 29, 362–372 (2004). Article CAS PubMed Google Scholar * van Nuland, A. J. M.
et al. GABAergic changes in the thalamocortical circuit in Parkinson’s disease. _Hum. Brain Mapp._ 41, 1017–1029 (2020). Article PubMed Google Scholar * Gibb, W. R. & Lees, A. J. The
relevance of the Lewy body to the pathogenesis of idiopathic Parkinson’s disease. _J. Neurol. Neurosurg. Psychiatry_ 51, 745–752 (1988). Article CAS PubMed PubMed Central Google Scholar
* Goetz, C. G. et al. Movement Disorder Society-sponsored revision of the Unified Parkinson’s Disease Rating Scale (MDS-UPDRS): process, format, and clinimetric testing plan. _Mov.
Disord._ 22, 41–47 (2007). Article PubMed Google Scholar * Bourgeois-Marcotte, J., Flamand-Roze, C., Denier, C. & Monetta, L. LAST-Q: adaptation et normalisation franco-québécoises du
Language Screening Test. _Rev. Neurol._ 171, 433–436 (2015). Article CAS PubMed Google Scholar * Radloff, L. S. The CES-D Scale. _Appl. Psychol. Meas._ 1, 385–401 (1977). Article
Google Scholar * Tomlinson, C. L. et al. Systematic review of levodopa dose equivalency reporting in Parkinson’s disease. _Mov. Disord._ 25, 2649–2653 (2010). Article PubMed Google
Scholar * Voon, V. & Fox, S. H. Medication-related impulse control and repetitive behaviors in Parkinson disease. _Arch. Neurol._ 64, 1089 (2007). Article PubMed Google Scholar *
Weintraub, D., David, A. S., Evans, A. H., Grant, J. E. & Stacy, M. Clinical spectrum of impulse control disorders in Parkinson’s disease. _Mov. Disord._ 30, 121–127 (2015). Article CAS
PubMed Google Scholar * Weintraub, D. et al. Questionnaire for impulsive-compulsive disorders in Parkinson’s Disease-Rating Scale. _Mov. Disord._ 27, 242–247 (2012). Article PubMed
Google Scholar * Patton, J. H., Stanford, M. S. & Barratt, E. S. Factor structure of the Barratt impulsiveness scale. _J. Clin. Psychol._ 51, 768–774 (1995). Article CAS PubMed
Google Scholar * Segal, D. L. In _The Corsini Encyclopedia of Psychology_ (eds. Weiner, I. B. & Craighead, W.) 1–3 (American Cancer Society, 2010). * Fabbrini, G., Juncos, J.,
Mouradian, M. M., Serrati, C. & Chase, T. N. Levodopa pharmacokinetic mechanisms and motor fluctuations in Parkinson’s disease. _Ann. Neurol._ 21, 370–376 (1987). Article CAS PubMed
Google Scholar * Tompson, D. & Oliver-Willwong, R. Pharmacokinetic and pharmacodynamic comparison of ropinirole 24-h prolonged release and ropinirole immediate release in patients with
Parkinson’s disease. _Clin. Neuropharmacol._ 32, 140–148 (2009). Article CAS PubMed Google Scholar * Hay, K. R. et al. Symptoms of medication withdrawal in Parkinson’s disease:
considerations for informed consent in patient-oriented research. _Pharm. Med._ 35, 163–167 (2021). Article Google Scholar * Mullins, P. G. et al. Current practice in the use of MEGA-PRESS
spectroscopy for the detection of GABA. _Neuroimage_ 86, 43–52 (2014). Article CAS PubMed Google Scholar * Edden, R. A. E., Puts, N. A. J., Harris, A. D., Barker, P. B. & Evans, C.
J. Gannet: A batch-processing tool for the quantitative analysis of gamma-aminobutyric acid-edited MR spectroscopy spectra. _J. Magn. Reson. Imaging_ 40, 1445–1452 (2014). Article PubMed
Google Scholar * Porges, E. C. et al. Impact of tissue correction strategy on GABA-edited MRS findings. _Neuroimage_ 162, 249–256 (2017). Article PubMed Google Scholar * Harris, A. D.,
Puts, N. A. J. & Edden, R. A. E. Tissue correction for GABA-edited MRS: considerations of voxel composition, tissue segmentation, and tissue relaxations. _J. Magn. Reson. Imaging_ 42,
1431–1440 (2015). Article PubMed PubMed Central Google Scholar Download references ACKNOWLEDGEMENTS This study was supported by the National Institutes of Health/National Institute of
Neurological Disorders and Stroke (R01NS097783, K23NS080988); the American Heart Association (14GRNT20150004); and the Clinical and Translational Science Awards award No. UL1TR000445 from
the National Center for Advancing Translational Science. AUTHOR INFORMATION AUTHORS AND AFFILIATIONS * Neurology, Vanderbilt University Medical Center, Nashville, TN, USA Paula Trujillo,
Alexander K. Song, Kaitlyn R. Hay, Megan Aumann, Manus J. Donahue & Daniel O. Claassen * Biostatistics, Vanderbilt University Medical Center, Nashville, TN, USA Yan Yan & Hakmook
Kang * Psychiatry and Behavioral Sciences, Vanderbilt University Medical Center, Nashville, TN, USA Manus J. Donahue Authors * Paula Trujillo View author publications You can also search for
this author inPubMed Google Scholar * Alexander K. Song View author publications You can also search for this author inPubMed Google Scholar * Kaitlyn R. Hay View author publications You
can also search for this author inPubMed Google Scholar * Megan Aumann View author publications You can also search for this author inPubMed Google Scholar * Yan Yan View author publications
You can also search for this author inPubMed Google Scholar * Hakmook Kang View author publications You can also search for this author inPubMed Google Scholar * Manus J. Donahue View
author publications You can also search for this author inPubMed Google Scholar * Daniel O. Claassen View author publications You can also search for this author inPubMed Google Scholar
CONTRIBUTIONS P.T.: involved in data acquisition, analysis and interpretation of data, and manuscript drafting. A.K.S.: involved in data acquisition, analysis and interpretation of data, and
manuscript revision. K.R.H.: involved in data acquisition, analysis and interpretation of data, and manuscript revision. M.A.: involved in analysis and interpretation of data, and
manuscript revision. Y.Y.: involved in statistical analysis and manuscript revision. H.K.: involved in statistical analysis and manuscript revision. M.J.D.: involved in study conception and
design, data acquisition, analysis and interpretation of data, and manuscript revision. D.O.C.: involved in study conception and design, data acquisition, analysis and interpretation of
data, and manuscript drafting and revision. CORRESPONDING AUTHOR Correspondence to Daniel O. Claassen. ETHICS DECLARATIONS COMPETING INTERESTS The authors declare no potential conflicts of
interest with respect to the research, authorship, and/or publication of this article. P.T. is a paid consultant for Alterity. M.J.D. is a paid consultant for Pfizer, Global Blood
Therapeutics, Spark Therapeutics, LymphaTouch, and Alterity and has served on advisory boards for Novartis and bluebird bio. He receives research grant funding from the National Institutes
of Health and Pfizer, research-related support from Philips Healthcare, and is the CEO of Biosight LLC which operates as a contracted Clinical Research Organization. D.O.C. receives research
support from the Griffin Foundation, the Huntington’s Disease Society of America, the National Institute of Neurological Disorders and Stroke, National Institute on Aging, National Center
for Complementary and Integrative Health, and Department of Defense; he received pharmaceutical grant support from AbbVie, Acadia, Biogen, BMS, Cerecour, Eli Lilly, Genetech/Roche, PTC
Therapeutics, Lundbeck, Jazz Pharmaceuticals, Teva Neuroscience, Spark, Wave Life Sciences, and Vaccinex; currently receives grant support from Genentech/ Roche, AbbVie, Prilenia, Alterity,
AbbVie, and CHDI, and he has served as a consultant to or on the advisory board of Acadia, Alterity, Adamas, Lundbeck, Neurocrine, Photopharmics, and Teva Neuroscience. ADDITIONAL
INFORMATION PUBLISHER’S NOTE Springer Nature remains neutral with regard to jurisdictional claims in published maps and institutional affiliations. SUPPLEMENTARY INFORMATION SUPPLEMENTARY
FIGURE 1 REPORTING SUMMARY CHECKLIST RIGHTS AND PERMISSIONS OPEN ACCESS This article is licensed under a Creative Commons Attribution 4.0 International License, which permits use, sharing,
adaptation, distribution and reproduction in any medium or format, as long as you give appropriate credit to the original author(s) and the source, provide a link to the Creative Commons
license, and indicate if changes were made. The images or other third party material in this article are included in the article’s Creative Commons license, unless indicated otherwise in a
credit line to the material. If material is not included in the article’s Creative Commons license and your intended use is not permitted by statutory regulation or exceeds the permitted
use, you will need to obtain permission directly from the copyright holder. To view a copy of this license, visit http://creativecommons.org/licenses/by/4.0/. Reprints and permissions ABOUT
THIS ARTICLE CITE THIS ARTICLE Trujillo, P., Song, A.K., Hay, K.R. _et al._ Dopamine-induced changes to thalamic GABA concentration in impulsive Parkinson disease patients. _npj Parkinsons
Dis._ 8, 37 (2022). https://doi.org/10.1038/s41531-022-00298-8 Download citation * Received: 23 July 2021 * Accepted: 01 February 2022 * Published: 05 April 2022 * DOI:
https://doi.org/10.1038/s41531-022-00298-8 SHARE THIS ARTICLE Anyone you share the following link with will be able to read this content: Get shareable link Sorry, a shareable link is not
currently available for this article. Copy to clipboard Provided by the Springer Nature SharedIt content-sharing initiative