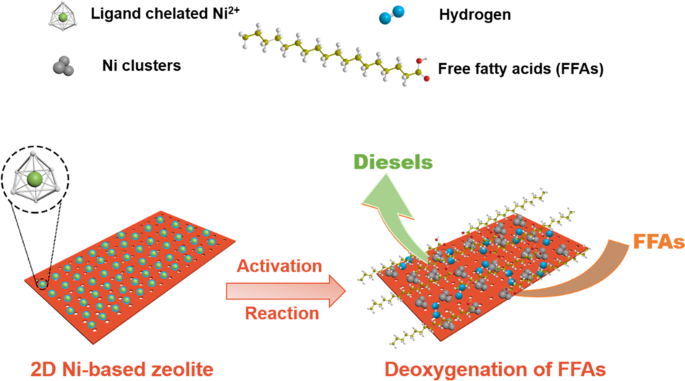
- Select a language for the TTS:
- UK English Female
- UK English Male
- US English Female
- US English Male
- Australian Female
- Australian Male
- Language selected: (auto detect) - EN
Play all audios:
ABSTRACT Deoxygenation of bioderived lipids into renewable transportation fuels is a promising route to decreasing the dependence on fossil sources. Ni-based catalysts are high performing
and cost-effective in deoxygenation reactions but suffer from severe sintering and aggregation. Herein, a ligand-chelating impregnation method was used to prepare highly dispersed Ni
nanoclusters on a two-dimensional (2D) ITQ-2 zeolite. Comprehensive characterization was utilized to monitor the changes in the organometallic precursors during activation and to investigate
their impact on the dispersion of the Ni nanoclusters on the ITQ-2 zeolite. The high external surface area and abundant surface defects of the 2D support enhanced the dispersion and
immobilization of the Ni nanoclusters and outperformed conventional zeolites. The protection of the Ni2+ cations by the organic ligand suppressed the aggregation of Ni species during the
activation processes, thereby leading to the formation of uniformly distributed Ni nanoclusters on the ITQ-2 zeolite. Due to the highly dispersed Ni nanoclusters and immobilization on the 2D
zeolite, the Ni/ITQ-2-co material prepared by the ligand-chelating impregnation approach showed outstanding activity and stability for conversions of stearic acid or palm oil to diesel
range alkanes. This work provides a rational design and precise modulation of metal-based catalysts for the production of renewable diesel. SIMILAR CONTENT BEING VIEWED BY OTHERS
METAL-ORGANIC FRAMEWORKS-DERIVED CAO/ZNO COMPOSITES AS STABLE CATALYSTS FOR BIODIESEL PRODUCTION FROM SOYBEAN OIL AT ROOM TEMPERATURE Article Open access 29 January 2025 HYDROGENATION OF
PYROLYSIS GASOLINE BY NOVEL NI-DOPED MOF DERIVED CATALYSTS FROM ZIF-8 AND ZIF-67 Article Open access 12 November 2022 (CO/ZN) AL2O4 NANO CATALYST FOR WASTE COOKING OIL CATALYTIC CRACKING
Article Open access 23 April 2022 INTRODUCTION The dwindling stores of fossil fuels and the escalating environmental problems arising from their overuse have made it urgent to develop
alternative sustainable energy sources. Biomass-derived triglycerides (TGs) are promising candidates and have been extensively utilized to produce liquid fuels due to their abundances,
renewability, eco-friendliness, and high energy densities1,2,3. The transesterification reaction between a TG and an alcohol over an acid or base catalyst has been commercialized around the
world to produce fatty acid alkyl esters (FAMEs), which are viewed as first-generation biodiesel4. Despite the environmental benefits, high flash point, and excellent lubricity, there are
drawbacks of FAMEs that severely limit extensive utilization, such as low oxidative stability, weak cold flow properties, and high residual oxygen content5. To overcome the disadvantages of
FAMEs, catalytic deoxygenation processes (hydrodeoxygenation, decarbonylation, and decarboxylation) have been developed to produce diesel-range alkanes by removing oxygen from the free fatty
acids (FFAs) or TGs6,7,8. Sulfided metal catalysts, such as sulfided nickel and molybdenum9,10, show high catalytic performance in the conversion of TGs to diesel-range alkanes. However,
sulfur leaching from catalysts can cause catalyst deactivation and diesel product contamination. Various supported metal catalysts have shown significant potential for use in the
deoxygenation reactions of TGs or FFAs to produce diesel-range alkanes, such as Ru11, Pt12, Pd13, Ni14, Co15, Cu14, etc. Support materials enhance the distributions and stabilities of the
metal sites and promote the catalytic capabilities of metal-based catalysts16. Zeolites with orderly distributed micropores, unique shape selectivities, high stabilities, and tunable
acidic/basic sites are promising supports for metals17,18,19,20,21. Zeolite-supported metal catalysts (metal/zeolite) have gained tremendous research interest for use in deoxygenation
reactions and other biomass upgrading processes22,23,24. Among these catalysts, Ni/zeolite catalysts are highly promising, owing to their sufficient activity and low costs7. Impregnation,
ion exchange, and deposition-precipitation are the most commonly used approaches for loading Ni onto zeolite supports, but their disadvantages, such as low dispersion, limited concentration
of exchangeable sites, and complex operations, must be overcome25,26. Zhao and her coauthors prepared core-shell structured Ni/Beta and hierarchical Ni/HUSY zeolites using a two-step
dissolution–recrystallization process and a post-treatment strategy, respectively6,7. Both of them exhibited high catalytic activity in the deoxygenation reactions of TGs or FFAs. However,
high Ni loadings (≥ 10 wt.%) on the zeolites are needed for the deoxygenation reactions, and the abundant Ni clusters are prone to sintering and aggregation during the activation and
reaction processes. Recently, Li et al. prepared ultrasmall Co clusters on H-ZSM-5 zeolite by grafting cobaltocene to the Brønsted acid sites of zeolites27. ZSM-5 zeolites with
three-dimensional micropores suffer from severe diffusion limitations for bulky molecules, such as TGs and FFAs. Therefore, it is highly desirable to prepare robust Ni/zeolites that
integrate stable and highly dispersed metal clusters with excellent mass transfer efficiencies on the zeolites for deoxygenation reactions of TGs and other bulky compounds. In this work,
highly dispersed Ni nanoclusters were distributed on 2D ITQ-2 zeolite, which possesses an open framework structure and high external surface area, and a facile impregnation strategy was used
that involves organometallic chemistry and is hereafter referred to as “ligand-chelating impregnation”. The cyclic organic amine (1,4,7,10-tetraazacyclododecane, abbreviated as
cyclen)-chelated Ni2+ cation was used as a precursor. A number of characterization techniques, including electron microscopy, spectroscopic analyses, temperature-programmed hydrogen
reduction (H2-TPR), and thermogravimetry analysis (TG), were used to investigate the effects of the ligand-chelated metal precursors and the microenvironments on the zeolite support
providing immobilization and dispersion of the Ni species. The prepared Ni/zeolites were used for deoxygenation of bulky biomass-derived stearic acid (SA) or palm oil to produce diesel-range
alkanes, which are regarded as promising renewable transportation fuels. The 2D Ni/ITQ-2-co zeolite prepared by the ligand-chelating impregnation method showed a higher catalytic rate than
the Ni/Y analog, with over a 10-fold improvement. In addition, due to immobilization by the 2D zeolite, the Ni/ITQ-2-co catalyst displayed outstanding stability during the deoxygenation
reactions. This facile synthetic strategy can be used to prepare other robust metal-based catalysts for complicated biomass conversions. MATERIALS AND METHODS The zeolite supports (Y,
MCM-22, and ITQ-2) were prepared with previously reported methods28,29,30. The Ni species was loaded on the zeolite with a conventional impregnation method and the ligand-chelating
impregnation strategy. The materials and chemicals used in this work and the synthetic methods, characterization techniques, and catalytic tests are described in detail in the Supplementary
Information. RESULTS AND DISCUSSION PHYSICOCHEMICAL PROPERTIES OF THE NI/ZEOLITE MATERIALS The X-ray diffraction (XRD) patterns for the prepared samples are shown in Fig. 1a and Fig. S1. The
typical diffraction peaks for Y, MCM-22, and ITQ-2 zeolites are observed in Fig. S128,29. There were no other peaks for amorphous or competing phases appearing in these XRD patterns. The
XRD pattern for the Ni/zeolite material is given in Fig. 1a, and the intensities of diffraction peaks for the Ni/zeolites were hardly changed after loading of the Ni species and subsequent
activation, indicating that the zeolite framework was preserved. N2 adsorption/desorption isotherms and the porosity parameters of the prepared Ni/zeolite materials are shown in Fig. 1b and
summarized in Table S1, respectively. Unlike Ni/Y (with a type-I isotherm implying a microporous structure), the Ni/ITQ-2 and Ni/MCM-22 samples displayed type-IV isotherms with a second
uptake at a relative pressure (_p_/_p_0) between 0.8 and 1.0, indicating the presence of intercrystalline meso-/macropores in the zeolite support. Ni/Y had the largest total surface area
(Stotal, 604 m2·g−1) and micropore volume (Vmicro, 0.26 cm3·g−1) among the prepared Ni/zeolites. The laminar Ni/MCM-22 sample showed a larger external surface area (Sext, 147 m2·g−1) than
Ni/Y (57 m2·g−1). 2D Ni/ITQ-2 and Ni/ITQ-2-co displayed large Sext values (431 and 415 m2·g−1, respectively) owing to the open structure of the 2D zeolite. The pore size distributions of the
Ni/zeolite samples are given in Fig. S2. Apparent mesopore distributions were observed for the laminar and 2D Ni/zeolite samples, which could have resulted from aggregation of the
interlayer mesopores. Other physicochemical properties of the prepared Ni/zeolites are also compiled in Table S1. An ICP‒OES analysis showed comparable Ni loadings (~2.3 wt.%) for the
prepared samples. The ratio of silicon to aluminum (Si/Al) was 16.7 for the laminar sample and 20.0 for the 2D Ni/zeolites. The morphologies of the zeolite supports were determined with
scanning electron microscopy (SEM). As shown in Fig. S3, the Y zeolite showed a pyramid-like morphology with an average particle size of ~1 μm. Curved and soft stacked nanosheets were
observed for the MCM-22 (Fig. S3b) and ITQ-2 (Fig. S3c) samples. After loading of the Ni clusters, the morphologies of the zeolite supports hardly changed (Fig. S4), indicating that the
zeolite supports were stable during the impregnation treatment. The effects of the zeolite morphology and micro/nanostructure on the dispersion of the Ni clusters was monitored by TEM
analysis. As shown in Fig. 1c–f, the average sizes of Ni clusters in the prepared Ni/zeolite samples were approximately 3 to 24 nm, which were much larger than the micropore size of each
zeolite, suggesting that the Ni clusters were mainly distributed on the external surfaces of the supports. Significantly, the particle sizes of the Ni clusters varied with the
micro/nanostructure of each zeolite support. The 2D ITQ-2 zeolite with the larger external surface area supported smaller Ni clusters than the 3D and laminar zeolites. The average sizes of
the Ni nanoparticles in the catalysts decreased in the order Ni/Y > Ni/MCM-22 > Ni/ITQ-2 > Ni/ITQ-2-co. Compared with the 3D and laminar zeolite supports, the average Ni
nanoparticle sizes of Ni/ITQ-2 and Ni/ITQ-2-co were as small as 6.4 ± 1.4 nm and 3.8 ± 0.6 nm, respectively. The small Ni clusters on the 2D ITQ-2 zeolite implied that the larger external
surface area favored dispersion of the metal particles. For the same 2D ITQ-2 support, the Ni clusters uniformly immobilized on Ni/ITQ-2-co were smaller than those on Ni/ITQ-2, suggesting
that the introduction of an organic ligand to chelate the Ni precursor prior to impregnation played an essential role in immobilizing the metal species and increasing their dispersion during
calcination and the subsequent reduction processes. In addition, energy-dispersive X-ray spectroscopy (STEM-EDS) was used to determine the distributions of the elements in the Ni/ITQ-2 and
Ni/ITQ-2-co samples (Fig. S5). The STEM-EDS images showed that the elemental Ni was well distributed on the ITQ-2 support. However, compared with the uniformly distributed Ni clusters on
Ni/ITQ-2-co, some of the Ni clusters in Ni/ITQ-2 were obviously agglomerated, which was consistent with the TEM observations. 29Si MAS NMR spectra of the zeolite supports are shown in Fig.
2a. Two strong signals at −102 ppm to −107 ppm appeared in the spectrum of H-Y, which were assigned to Q4(1Al) and Q4(0Al) in the H-Y zeolite31. Moreover, the shoulder peak at −100 ppm
indicated the presence of minimal silanol species distributed on the external surface of the H-Y zeolite32. For the ITQ-2 and MCM-22 samples, the signals at −105 to −120 ppm corresponded to
the Q4 environments, while the Q3 T-sites (Si(OSi)3OH) exhibited peaks at ca. −100 ppm33,34. After deconvolution, the populations of the Q3 sites were 18% in ITQ-2 and 8% in MCM-22,
signifying that ITQ-2 had more Si-OH defect sites than MCM-22. Furthermore, the influence of Ni introduction into ITQ-2 at the Si-OH sites was studied by Fourier transform infrared
spectroscopy (FTIR). As shown in Fig. S6a, introduction of the Ni species into ITQ-2 resulted in decreases in the number of isolated Si−OH sites exhibiting bands that were centered at 3745
cm−1, implying that the defect sites (Si−OH) in ITQ-2 were covered by the Ni species. Therefore, based on the above analyses of the Si-OH sites by 29Si MAS NMR and FTIR, it can be deduced
that the abundant isolated Si−OH defects on ITQ-2 are probably effective in immobilizing the Ni species, thereby leading to better dispersion and smaller particle sizes for the Ni clusters
compared with those on the MCM-22 and Y zeolite supports. The above results were consistent with the TEM analysis (Fig. 1c‒f). The profiles for temperature-programmed desorption of ammonia
(NH3-TPD) from the prepared samples are given in Fig. 2b. An apparent desorption peak at 170–190 °C was observed for all of the samples, and this was ascribed to weakly acidic sites, such as
defect sites (Si-OH, etc.)35. The desorption peak at 335–350 °C was attributed to the desorption of ammonia from medium acid sites36. For the Ni/MCM-22 and Ni/ITQ-2(-co) samples, the
desorption peaks at approximately 420 °C corresponded to the desorption of ammonia from strong Brønsted acid sites37. The acidity of ITQ-2 was weaker than that of MCM-22, which may be
attributed to dealumination occurring during the swelling process28. Among the samples, the Ni/Y exhibited the highest acid concentration and strength (at 550 °C) and the lowest Si/Al ratio
and a unique framework structure. TG analyses of the as-synthesized Ni/ITQ-2 and Ni/ITQ-2-co samples (uncalcined) were conducted to investigate the effect of the chelating ligand on the Ni
species formed during calcination. The TG results showed that the mass-loss profiles of the uncalcined samples were affected by the presence of the cyclen ligand. As shown in Fig. 2c, in
addition to the mass loss seen at 120 °C, which corresponded to water removal from the two samples, stepwise mass losses from 120‒450 °C were observed for the Ni/ITQ-2-co samples; this
included a gradual loss at 120‒210 °C and two sharp losses at 210 and 420 °C, which implied stepwise decomposition of the Ni-cyclen complex on ITQ-2 during calcination. The organic species
in Ni/ITQ-2-co was completely removed at 500 °C in flowing air. In comparison, an apparent mass loss at 120‒210 °C for Ni/ITQ-2 indicated quick combustion of the nickel nitrate on ITQ-2
zeolite. The TG data for the uncalcined Ni/ITQ-2 and Ni/ITQ-2-co showed that Ni-cyclen was more stable during calcination than the bare Ni cations on the zeolite. Stepwise decomposition of
the Ni-cyclen complex could stabilize the Ni species and inhibit rapid aggregation during calcination, thereby improving the dispersion of NiOx on the ITQ-2 zeolite. The H2-TPR profiles for
the calcined NiOx/ITQ-2 and NiOx/ITQ-2-co are shown in Fig. 2d. A sharp peak at 450 °C and two small peaks centered at 355 °C and 560 °C were identified for these two samples. The
low-temperature peak at 355 °C was ascribed to the bulky NiOx particles distributed on the surface of ITQ-2, which were reduced at low temperatures38. NiOx/ITQ-2 exhibited higher profiles at
355 °C than NiOx/ITQ-2-co, indicating that bulkier NiOx clusters were formed on NiOx/ITQ-2 via aggregation of the Ni species during calcination. The sharp peak at 450 °C corresponded to the
highly dispersed NiOx that was strongly immobilized on the zeolite support, which resulted in the higher reduction temperature39. Compared with NiOx/ITQ-2, for which nickel nitrate was used
as the precursor, the NiOx/ITQ-2-co prepared by the ligand-chelating impregnation approach exhibited more highly dispersed NiOx clusters that were strongly immobilized on ITQ-2. These
H2-TPR results were confirmed by TEM analyses of the calcined NiOx/ITQ-2 and NiOx/ITQ-2-co samples. As shown in Fig. 3a, b, uniform NiOx clusters with an average size of 2.9 ± 0.8 nm were
more homogeneously distributed throughout the NiOx/ITQ-2-co sample (Fig. 3b), compared with NiOx/ITQ-2 with an average size of 4.8 ± 1.7 nm (Fig. 3a). These support-stabilized NiOx species
suppressed quick agglomeration of the NiOx species during reduction in H2, thereby efficiently improving the dispersion of the Ni clusters. The reduction peak at 560 °C was assigned to the
exchanged Ni2+ on the zeolite structure, which required a higher reduction temperature than NiOx40. Thus, the reductions for all of the prepared samples were performed at 560 °C. The nickel
species influenced by the organic ligand and the ITQ-2 support were characterized by ultraviolet‒visible diffuse reflectance spectroscopy (UV − Vis). The UV − Vis spectra of uncalcined and
calcined Ni/ITQ-2 and Ni/ITQ-2-co are shown in Fig. 3c, d. A single band at 210 nm was ascribed to the characteristic charge-transfer transition of the tetracoordinated aluminum in the
zeolite framework41. When neat Ni(NO3)3 was used as the precursor for impregnation on ITQ-2, UV spectral bands at 298, 400, and 655 nm were detected (Fig. 3c), and these were assigned to the
charge-transfer transition of NO3- with Ni2+ and the d–d transition of [Ni(H2O)6]2+ on the zeolites42,43. When the ITQ-2 zeolite was impregnated with the cyclen-chelated Ni2+ complex,
higher energy UV bands were observed compared to the Ni/ITQ-2 sample. As shown in Fig. 3d, three distinct bands were observed at 260, 362, and 565 nm. The band at 260 nm was ascribed to the
isolated distorted octahedral Ni2+ complexes that were immobilized on the zeolite support43. The presence of the isolated Ni2+ species suggested that cyclen chelation prevented aggregation
of the Ni2+ species impregnated on the zeolites. In addition, two bands at 362 and 565 nm corresponded to d–d transitions of the Ni2+ complex in Ni/ITQ-2-co. The higher energies of the Ni2+
d–d transition bands compared with those for Ni/ITQ-2 were caused by the presence of a strong organic ligand that coordinated the Ni2+ cations42. In addition, the effect of the interactions
between the Ni-cyclen complex and the ITQ-2 support on the distinct coordination environment identified by UV − Vis cannot be neglected. Several spectroscopic studies have demonstrated
strong interactions of organometallic complexes and acidic OH groups on the support surface27,44,45. The strong interactions of the Ni-cyclen complex with the acidic surface of the ITQ-2
after impregnation enabled uniform distribution of the Ni precursor. Thus, the use of a ligand-chelated Ni complex as the precursor in the impregnation process improved the molecular
interactions with the zeolite support and protected the active Ni species from agglomeration. The distributions of the organometallic complexes on the zeolites were also confirmed by FTIR
analyses. As shown in Fig. S6b, the uncalcined Ni/ITQ-2-co sample prepared by the ligand-chelating impregnation route showed two obvious C-H stretching vibrations at 2944 and 2894 cm−1,
which implied the presence of the Ni-cyclen complex on the ITQ-2 support. The FTIR results were consistent with the UV‒Vis analyses. These results showed the ligand enabled uniform
immobilization of the Ni species on the ITQ-2 zeolite by chelating the Ni cations in the precursor solution. A band at approximately 265 nm with a shoulder at 408 nm was observed in the UV
spectrum of NiOx/ITQ-2-co (Fig. 3d). However, NiOx/ITQ-2 exhibited two bands at 275 and 427 nm. These bands were ascribed to NiOx species, as reported in the literature46. Indeed, shifts of
NiOx bands toward higher wavelengths for NiOx/ITQ-2 indicated the NiOx particles were larger than those in NiOx/ITQ-2-co47, which was consistent with the average NiOx sizes determined in the
TEM analysis (Fig. 3b). The electronic states of the Ni species in the Ni/ITQ-2 and Ni/ITQ-2-co samples were determined by X-ray photoelectron spectroscopy (XPS), which was used to
investigate the interactions between metallic Ni (Ni0) and the ITQ-2 zeolite. The XPS spectra of Ni/ITQ-2 and Ni/ITQ-2-co are shown in Fig. S7. Deconvolution of the Ni 2p XPS peak resulted
in four peaks at ca. 855.4 or 856.5 eV for Ni0 (2p3/2), 862.1 eV for Ni2+ (2p3/2), 874.5 eV for Ni0 (2p1/2), and 880.8 eV for Ni2+ (2p1/2)48. Compared with the isolated nickel metal, which
exhibited a Ni0 (2p3/2) binding energy (BE) of 853 eV, the ITQ-2-supported nickel showed higher BEs (∼856 eV) for Ni0 (2p3/2) due to the electronic influence of the zeolite support49. It has
been reported that interactions of metal clusters with oxygens in the zeolite framework gave rise to positive charges on the metal surface and higher BEs for the metal nanoclusters50,51. As
shown in Fig. S7, Ni/ITQ-2 exhibited a main peak at 855.4 eV for Ni0 (2p3/2), while the Ni0 (2p3/2) peak was at 856.5 eV for Ni/ITQ-2-co. The higher BE for Ni0 in Ni/ITQ-2-co indicated that
the Ni nanoclusters loaded by the ligand-chelating impregnation method had stronger interactions with the ITQ-2 support relative to those seen after the conventional impregnation
approach48. This could have originated from more highly dispersed NiOx that was strongly stabilized on the ITQ-2 before reduction, as demonstrated by the TG and H2-TPR analyses provided
above. Furthermore, the strongly immobilized Ni nanoparticles on Ni/ITQ-2-co inhibited rapid aggregation during the reaction. CATALYTIC PERFORMANCE OF THE NI/ZEOLITE CATALYSTS The
deoxygenation reaction of SA was used as a model reaction for conversions of FFAs to diesel-range alkanes. The reaction routes for SA conversion are depicted in Scheme 1. SA could undergo
full hydrogenation of the carboxyl group to produce 1-octadecanol, which may be converted to octadecene by acid-catalyzed dehydration. Octadecene is easily hydrogenated at metal sites to
yield n-octadecane. In addition, partial hydrogenation of the carboxyl group would produce stearaldehyde, which could also be converted to n-heptadecane through decarbonylation.
Alternatively, the SA could also produce heptadecene directly via decarboxylation. In the present work, the catalytic activities of the prepared 3D and 2D Ni/zeolites were investigated with
the deoxygenation of SA (Fig. 4). The normalized reaction rate was calculated with Equation S1 to compare the catalytic activities for all of the Ni/zeolite materials prepared. As shown in
Fig. 4a, the normalized rates of the samples increased in the order Ni/Y < Ni/MCM-22 < Ni/ITQ-2 < Ni/ITQ-2-co. The 2D Ni/ITQ-2 catalyst exhibited a remarkably higher conversion rate
(90 gSA gNi−1 h−1) compared to those of the laminar Ni/MCM-22 (50 gSA gNi−1 h−1) and 3D Ni/Y (12 gSA gNi−1 h−1) samples. The enhanced activity of Ni/ITQ-2 was attributed to the open
structure and more accessible acid sites in 2D ITQ-2, which promoted dehydration and facilitated conversion of the SA. In addition, the abundant defect sites on the ITQ-2 surface aided the
dispersion of the Ni clusters on the ITQ-2 support. Thus, the highly dispersed Ni nanoparticles promoted deoxygenation reactions. Compared with Ni/ITQ-2, the Ni/ITQ-2-co sample prepared by
using Ni-cyclen as the precursor for impregnation showed a markedly higher rate (168 gSA gNi-1 h-1), which was consistent with the relative average sizes of the Ni nanoparticles. Despite the
comparable Ni loadings in the two 2D samples, the smaller sizes and higher dispersion of the Ni nanoparticles in Ni/ITQ-2-co provided more metal active sites for the deoxygenation of SA
than Ni/ITQ-2. The large external surface area, highly dispersed Ni nanoparticles, and abundant accessible active sites on Ni/ITQ-2-co reduced the diffusion limitation, provided more active
sites and showed superior activity in the deoxygenation reaction of SA compared to most reported catalysts (Table S2). The conversion of SA and the product distributions on Ni/ITQ-2 and
Ni/ITQ-2-co are shown in Fig. 4b, c. After reacting for 4 h, the Ni/ITQ-2-co catalyst afforded 98% conversion of the SA, which was much higher than that seen with Ni/ITQ-2 (70%). The main
products for both catalysts were n-octadecane (n-C18) and n-heptadecane (n-C17), suggesting that decarboxylation/decarbonylation occurred in addition to hydrodeoxygenation of the SA11. It is
worth noting that the ratios of n-C18 to n-C17 (~2.5 in molar) were comparable for Ni/ITQ-2 and Ni/ITQ-2-co, indicating that the SA deoxygenation reactions on Ni/ITQ-2 and Ni/ITQ-2-co used
the same pathway. Thus, the excellent catalytic performance of Ni/ITQ-2-co was attributed to highly dispersed Ni nanoparticles immobilized on ITQ-2 via the ligand-chelating impregnation
approach. To expand the substrate scope, the deoxygenation of palm oil, which is rich in hexadecanoic and octadecanoic acids, was carried out over Ni/ITQ-2-co. As shown in Fig. 4d, the main
products were n-C15–C18 alkanes, and the yield of diesel-range alkanes was 83 wt%, which was close to the theoretical yield of 85 wt%. After the reactions, the Ni/ITQ-2 and Ni/ITQ-2-co were
collected by centrifugation, washed with acetone, and dried overnight for regeneration. The activity of the Ni/ITQ-2-co catalyst was nearly unchanged after four consecutive cycles (57% to
53%, as shown in Fig. 4e), whereas the efficiency for conversion of SA over Ni/ITQ-2 was decreased to half of the initial efficiency after four cycles (Fig. 4f). To explain the different
stabilities, the Ni loadings of both catalysts were measured via ICP‒OES. The results showed slight decreases in the Ni loadings on Ni/ITQ-2 (2.33 wt%) and Ni/ITQ-2-co (2.51 wt%) after the
reaction (Table S1). This indicated that the 2D support with abundant defect sites and high external surface area facilitated immobilization of the Ni clusters during the reaction.
Furthermore, a TEM analysis was performed on the used Ni/ITQ-2 and Ni/ITQ-2-co to determine the sizes of the Ni nanoparticles after the reactions. As shown in Fig. S8, obvious aggregation of
the Ni clusters had occurred for Ni/ITQ-2 after the deoxygenation reaction of SA, which resulted in a significant increase in the average size of the Ni clusters from 6.4 nm to 17.0 nm. In
contrast, the average size of the Ni nanoparticles on Ni/ITQ-2-co had increased only slightly from 3.8 nm to 5.3 nm after the reaction. Therefore, the excellent stability of Ni/ITQ-2-co was
attributed to the stable Ni sites that resulted from the strong interactions with the ITQ-2 support, as indicated by the XPS analysis discussed above. The uniform distribution and strong
immobilization of Ni on the Ni/ITQ-2-co catalyst effectively suppressed migration of the Ni nanoclusters during the reaction and regeneration processes. Taken together, these findings
provide further support for the use of the ligand-chelating impregnation approach in preparing the Ni/ITQ-2-co catalyst. As illustrated in Scheme 2, the 2D zeolite support facilitated
distribution of the Ni species after impregnation, since its external surface area was larger than that of the 3D support (Scheme 2a). During the activation process, the cyclen-chelated Ni
complexes underwent stepwise decomposition, unlike the bare Ni2+ ions. This process suppressed rapid aggregation of the Ni species and formed highly dispersed Ni nanoclusters on the ITQ-2
zeolite (Scheme 2b). The ligand-chelating impregnation strategy stabilized the highly dispersed Ni nanoclusters on the ITQ-2 zeolite, and this involved protection of the Ni2+ by the
chelating ligand and immobilization on the 2D zeolite. Consequently, the ITQ-2-supported Ni nanoclusters displayed superior activity and stability during the conversions of palm oil or SA to
diesel-range alkanes (Scheme 2c). CONCLUSION In conclusion, a series of 2D and 3D Ni/zeolites was prepared via an impregnation approach and used in sustainable production of diesel fuel via
deoxygenation. The present work highlighted the advantages of utilizing a 2D zeolite support with a large external surface area and abundant defect sites, which were beneficial in
dispersing the Ni nanoparticles formed via the facile impregnation method. Compared to conventional 3D and laminar zeolite supports, the 2D ITQ-2 zeolite-supported Ni materials exhibited
markedly smaller Ni particle sizes and improved transport efficiencies for bulky reactants. Importantly, the ligand-chelated Ni complex used as the precursor for the impregnation process
provided smaller Ni nanoparticles and facilitated dispersion via a combination of ligand protection and strong interactions between the Ni species and the 2D ITQ-2 zeolite. Consequently, the
Ni/ITQ-2-co catalyst prepared by the ligand-chelating impregnation approach showed superior activity and stability in the deoxygenation reactions of SA or palm oil, and this offers a
promising solution for renewable production of diesel fuel. REFERENCES * Yusuf, N. N. A. N., Kamarudin, S. K. & Yaakub, Z. Overview on the current trends in biodiesel production. _Energy
Convers. Manag._ 52, 2741–2751 (2011). CAS Google Scholar * Pang, H., Yang, G., Li, L. & Yu, J. Efficient transesterification over two-dimensional zeolites for sustainable biodiesel
production. _Green. Energy Environ._ 5, 405–413 (2020). CAS Google Scholar * Amini, Z., Ilham, Z., Ong, H. C., Mazaheri, H. & Chen, W.-H. State of the art and prospective of
lipase-catalyzed transesterification reaction for biodiesel production. _Energy Convers. Manag._ 141, 339–353 (2017). CAS Google Scholar * Demirbas, A. Comparison of transesterification
methods for production of biodiesel from vegetable oils and fats. _Energy Convers. Manag._ 49, 125–130 (2008). CAS Google Scholar * Demirbas, A. Progress and recent trends in biodiesel
fuels. _Energy Convers. Manag._ 50, 14–34 (2009). CAS ADS Google Scholar * Ma, B., Cui, H. M., Wang, D. R., Wu, P. & Zhao, C. Controllable hydrothermal synthesis of Ni/H-BEA with a
hierarchical core-shell structure and highly enhanced biomass hydrodeoxygenation performance. _Nanoscale_ 9, 5986–5995 (2017). CAS PubMed Google Scholar * Ma, B., Yi, X. F., Chen, L.,
Zheng, A. M. & Zhao, C. Interconnected hierarchical HUSY zeolite-loaded Ni nano-particles probed for hydrodeoxygenation of fatty acids, fatty esters, and palm oil. _J. Mater. Chem. A_ 4,
11330–11341 (2016). CAS Google Scholar * Foraita, S. et al. Controlling hydrodeoxygenation of stearic acid to n-Heptadecane and n-Octadecane by adjusting the chemical properties of
Ni/SiO2-ZrO2 catalyst. _Chemcatchem_ 9, 195–203 (2017). CAS Google Scholar * Kubička, D. & Kaluža, L. Deoxygenation of vegetable oils over sulfided Ni, Mo and NiMo catalysts. _Appl.
Catal. A_ 372, 199–208 (2010). Google Scholar * Kubičková, I., Snåre, M., Eränen, K., Mäki-Arvela, P. & Murzin, D. Y. Hydrocarbons for diesel fuel via decarboxylation of vegetable oils.
_Catal. Today_ 106, 197–200 (2005). Google Scholar * Di, L. et al. Robust ruthenium catalysts for the selective conversion of stearic acid to diesel-range alkanes. _Appl. Catal. B_ 201,
137–149 (2017). CAS ADS Google Scholar * Janampelli, S. & Darbha, S. Selective and reusable Pt-WOx/Al2O3 catalyst for deoxygenation of fatty acids and their esters to diesel-range
hydrocarbons. _Catal. Today_ 309, 219–226 (2018). CAS Google Scholar * Hengsawad, T., Jindarat, T., Resasco, D. E. & Jongpatiwut, S. Synergistic effect of oxygen vacancies and highly
dispersed Pd nanoparticles over Pd-loaded TiO2 prepared by a single-step sol–gel process for deoxygenation of triglycerides. _Appl. Catal. A_ 566, 74–86 (2018). CAS Google Scholar * Cheah,
K. W. et al. Monometallic and bimetallic catalysts based on Pd, Cu and Ni for hydrogen transfer deoxygenation of a prototypical fatty acid to diesel range hydrocarbons. _Catal. Today_ 355,
882–892 (2020). CAS Google Scholar * Kamaruzaman, M. F., Taufiq-Yap, Y. H. & Derawi, D. Green diesel production from palm fatty acid distillate over SBA-15-supported nickel, cobalt,
and nickel/cobalt catalysts. _Biomass-. Bioenergy_ 134, 105476 (2020). CAS Google Scholar * Song, W. J., Liu, Y. S., Barath, E., Zhao, C. & Lercher, J. A. Synergistic effects of Ni and
acid sites for hydrogenation and C-O bond cleavage of substituted phenols. _Green. Chem._ 17, 1204–1218 (2015). CAS Google Scholar * Liu, S. et al. Cascade adsorptive separation of light
hydrocarbons by commercial zeolites. _J. Energy Chem._ 72, 299–305 (2022). CAS Google Scholar * Di, J., Li, L., Wang, Q. & Yu, J. Porous membranes with special wettabilities: designed
fabrication and emerging application. _CCS Chem._ 3, 2280–2297 (2021). CAS Google Scholar * Chai, Y., Dai, W., Wu, G., Guan, N. & Li, L. Confinement in a zeolite and zeolite catalysis.
_Acc. Chem. Res._ 54, 2894–2904 (2021). CAS PubMed Google Scholar * Yu, J. & Zhao, D. Preface to special topic on new era of zeolite science. _Natl Sci. Rev._ 9, nwac157 (2022).
PubMed PubMed Central Google Scholar * Shi, T. et al. Scalable synthesis of ultrastable lead halide perovskite-zeolite composites via a chemical vapor method in air. _NPG Asia Mater._ 14,
87 (2022). CAS ADS Google Scholar * Sudarsanam, P., Peeters, E., Makshina, E. V., Parvulescu, V. I. & Sels, B. F. Advances in porous and nanoscale catalysts for viable biomass
conversion. _Chem. Soc. Rev._ 48, 2366–2421 (2019). CAS PubMed Google Scholar * Luo, W. et al. Zeolite-supported metal catalysts for selective hydrodeoxygenation of biomass-derived
platform molecules. _Green. Chem._ 21, 3744–3768 (2019). CAS Google Scholar * Shi, Y. et al. Recent progress on upgrading of bio-oil to hydrocarbons over metal/zeolite bifunctional
catalysts. _Catal. Sci. Technol._ 7, 2385–2415 (2017). CAS Google Scholar * Wang, N., Sun, Q. & Yu, J. Ultrasmall metal nanoparticles confined within crystalline nanoporous materials:
a fascinating class of nanocatalysts. _Adv. Mater._ 31, 1803966 (2019). Google Scholar * Song, W. J., Zhao, C. & Lercher, J. A. Importance of size and distribution of Ni nanoparticles
for the hydrodeoxygenation of microalgae oil. _Chem. Eur. J._ 19, 9833–9842 (2013). CAS PubMed Google Scholar * Wu, G., Zhang, N., Dai, W., Guan, N. & Li, L. Construction of
bifunctional Co/H-ZSM-5 catalysts for the hydrodeoxygenation of stearic acid to diesel-range alkanes. _ChemSusChem_ 11, 2179–2188 (2018). CAS PubMed Google Scholar * Corma, A., Fornes,
V., Pergher, S. B., Maesen, T. L. M. & Buglass, J. G. Delaminated zeolite precursors as selective acidic catalysts. _Nature_ 396, 353 (1998). CAS ADS Google Scholar * Oleksiak, M. D.
et al. Organic-free synthesis of a highly siliceous Faujasite zeolite with spatially biased Q(4)(nAl) Si speciation. _Angew. Chem. Int. Ed._ 56, 13366–13371 (2017). CAS Google Scholar *
Pang, H., Yang, G., Li, L. & Yu, J. Esterification of oleic acid to produce biodiesel over 12-tungstophosphoric acid anchored two-dimensional zeolite. _Chem. Res. Chin. Univ_. 37,
1072–1078 (2021). * Yan, Z. et al. On the acid-dealumination of USY zeolite: a solid state NMR investigation. _J. Mol. Catal. A: Chem._ 194, 153–167 (2003). CAS Google Scholar * Zhang, L.,
Chen, K., Chen, B., White, J. L. & Resasco, D. E. Factors that determine zeolite stability in hot liquid water. _J. Am. Chem. Soc._ 137, 11810–11819 (2015). CAS PubMed Google Scholar
* Corma, A. et al. Characterization and catalytic activity of MCM-22 and MCM-56 compared with ITQ-2. _J. Catal._ 191, 218–224 (2000). CAS Google Scholar * Machado, V., Rocha, J.,
Carvalho, A. P. & Martins, A. Modification of MCM-22 zeolite through sequential post-synthesis treatments. Implications on the acidic and catalytic behaviour. _Appl. Catal. A_ 445-446,
329–338 (2012). CAS Google Scholar * Qiu, Z. et al. Tailoring the local environment of silver in SSZ-13 zeolites for low-temperature catalytic oxidation of ammonia. _Appl. Surf. Sci._ 598,
153856 (2022). CAS Google Scholar * Unverricht, S., Hunger, M., Ernst, S., Karge, H. G. & Weitkamp, J. Zeolite MCM-22: synthesis, dealumination and structural characterization. _Stud.
Surf. Sci. Catal._ 84, 37–44 (1994). CAS Google Scholar * Shu, Y., Ma, D., Xu, L., Xu, Y. & Bao, X. Methane dehydro‐aromatization over Mo/MCM‐22 catalysts: a highly selective catalyst
for the formation of benzene. _Catal. Lett._ 70, 67–73 (2000). CAS Google Scholar * Chen, B.-H. et al. Towards a full understanding of the nature of Ni(ii) species and hydroxyl groups
over highly siliceous HZSM-5 zeolite supported nickel catalysts prepared by a deposition–precipitation method. _Dalton T_ 45, 2720–2739 (2016). CAS Google Scholar * Bacariza, M. C., Amjad,
S., Teixeira, P., Lopes, J. M. & Henriques, C. Boosting Ni dispersion on zeolite-supported catalysts for CO2 methanation: the influence of the impregnation solvent. _Energy Fuels_ 34,
14656–14666 (2020). CAS Google Scholar * Bacariza, M. C. et al. CO2 hydrogenation over Ni-based zeolites: effect of catalysts preparation and pre-reduction conditions on methanation
performance. _Top. Catal._ 59, 314–325 (2016). CAS Google Scholar * Zanjanchi, M. A. & Razavi, A. Identification and estimation of extra-framework aluminium in acidic mazzite by
diffuse reflectance spectroscopy. _Spectrochim. Acta, Part A_ 57, 119–127 (2001). CAS ADS Google Scholar * Espinosa-Alonso, L., de Jong, K. P. & Weckhuysen, B. M. Effect of the Nickel
precursor on the impregnation and drying of γ-Al2O3 catalyst bodies: A UV−vis and IR microspectroscopic study. _J. Phys. Chem. C._ 112, 7201–7209 (2008). CAS Google Scholar * Hass, E. C.
& Plath, P. J. UV-visible transmission microscope spectrophotometry on x-type zeolites: Part I. Formation of different nickel complexes in zeolite X. _J. Mol. Catal._ 14, 35–52 (1982).
CAS Google Scholar * Négrier, F. et al. A systematic study of the interactions between chemical partners (metal, ligands, counterions, and support) involved in the design of
Al2O3-supported nickel catalysts from Diamine−Ni(II) Chelates. _J. Phys. Chem. B_ 109, 2836–2845 (2005). PubMed Google Scholar * Sun, K.-Q., Marceau, E. & Che, M. Evolution of nickel
speciation during preparation of Ni–SiO2 catalysts: effect of the number of chelating ligands in [Ni(en)x(H2O)6−2x]2+ precursor complexes. _Phys. Chem. Chem. Phys._ 8, 1731–1738 (2006). CAS
PubMed Google Scholar * Qi, Y., Qi, H., Li, J. & Lu, C. Synthesis, microstructures and UV–vis absorption properties of β-Ni(OH)2 nanoplates and NiO nanostructures. _J. Cryst. Growth_
310, 4221–4225 (2008). CAS ADS Google Scholar * Bacariza, M. C., Maleval, M., Graça, I., Lopes, J. M. & Henriques, C. Power-to-methane over Ni/zeolites: Influence of the framework
type. _Microporous Mesoporous Mater._ 274, 102–112 (2019). CAS Google Scholar * Zahmakıran, M. et al. Zeolite framework stabilized nickel(0) nanoparticles: active and long-lived catalyst
for hydrogen generation from the hydrolysis of ammonia-borane and sodium borohydride. _Catal. Today_ 170, 76–84 (2011). Google Scholar * Kumari, S. & Ray, S. Zeolite encapsulated Ni(ii)
Schiff-base complexes: improved catalysis and site isolation. _N. J. Chem._ 44, 14953–14963 (2020). CAS Google Scholar * Guczi, L. & Bazin, D. Structure and selectivity of metal
catalysts: revisiting bimetallic zeolite systems. _Appl. Catal. A_ 188, 163–174 (1999). CAS Google Scholar * Fukuoka, A. et al. Preparation, XAFS characterization, and catalysis of
platinum nanowires and nanoparticles in mesoporous silica FSM-16. _Top. Catal._ 18, 73–78 (2002). CAS Google Scholar Download references ACKNOWLEDGEMENTS This work is supported by the
National Natural Science Foundation of China (Grant Nos. 22288101, 21835002, and 22001090) and the 111 Project (B17020). Yang acknowledges the Department of Science and Technology of Jilin
Province (Grant 20200201096JC). AUTHOR INFORMATION AUTHORS AND AFFILIATIONS * State Key Laboratory of Inorganic Synthesis and Preparative Chemistry, College of Chemistry, Jilin University,
2699 Qianjin Street, Changchun, 130012, P. R. China Hao Pang, Guoju Yang & Jihong Yu * International Center of Future Science, Jilin University, 2699 Qianjin Street, Changchun, 130012,
P. R. China Hao Pang & Jihong Yu * Electron Microscopy Center, Jilin University, 2699 Qianjin Street, Changchun, 130012, P. R. China Lin Li Authors * Hao Pang View author publications
You can also search for this author inPubMed Google Scholar * Guoju Yang View author publications You can also search for this author inPubMed Google Scholar * Lin Li View author
publications You can also search for this author inPubMed Google Scholar * Jihong Yu View author publications You can also search for this author inPubMed Google Scholar CONTRIBUTIONS J.Y.
and G.Y. designed the experiments and prepared the manuscript. H.P. and G.Y. conducted the syntheses, characterizations, and catalyst evaluations. All authors discussed the results and
commented on the manuscript. CORRESPONDING AUTHORS Correspondence to Guoju Yang or Jihong Yu. ETHICS DECLARATIONS COMPETING INTERESTS The authors declare no competing interests. ADDITIONAL
INFORMATION PUBLISHER’S NOTE Springer Nature remains neutral with regard to jurisdictional claims in published maps and institutional affiliations. SUPPLEMENTARY INFORMATION ELECTRONIC
SUPPLEMENTARY INFORMATION RIGHTS AND PERMISSIONS OPEN ACCESS This article is licensed under a Creative Commons Attribution 4.0 International License, which permits use, sharing, adaptation,
distribution and reproduction in any medium or format, as long as you give appropriate credit to the original author(s) and the source, provide a link to the Creative Commons license, and
indicate if changes were made. The images or other third party material in this article are included in the article’s Creative Commons license, unless indicated otherwise in a credit line to
the material. If material is not included in the article’s Creative Commons license and your intended use is not permitted by statutory regulation or exceeds the permitted use, you will
need to obtain permission directly from the copyright holder. To view a copy of this license, visit http://creativecommons.org/licenses/by/4.0/. Reprints and permissions ABOUT THIS ARTICLE
CITE THIS ARTICLE Pang, H., Yang, G., Li, L. _et al._ Toward the production of renewable diesel over robust Ni nanoclusters highly dispersed on a two-dimensional zeolite. _NPG Asia Mater_
15, 24 (2023). https://doi.org/10.1038/s41427-023-00471-2 Download citation * Received: 03 August 2022 * Revised: 21 February 2023 * Accepted: 24 February 2023 * Published: 21 April 2023 *
DOI: https://doi.org/10.1038/s41427-023-00471-2 SHARE THIS ARTICLE Anyone you share the following link with will be able to read this content: Get shareable link Sorry, a shareable link is
not currently available for this article. Copy to clipboard Provided by the Springer Nature SharedIt content-sharing initiative