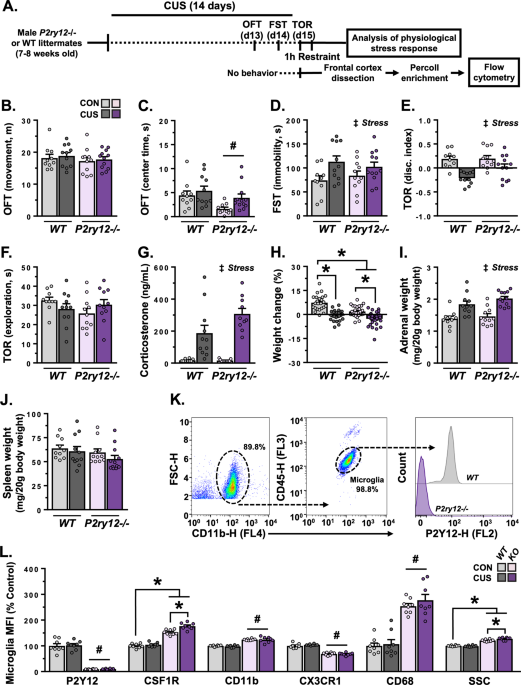
- Select a language for the TTS:
- UK English Female
- UK English Male
- US English Female
- US English Male
- Australian Female
- Australian Male
- Language selected: (auto detect) - EN
Play all audios:
ABSTRACT Chronic unpredictable stress (CUS) drives microglia-mediated neuronal remodeling and synapse loss in the prefrontal cortex (PFC), contributing to deficits in cognition and behavior.
However, it remains unclear what mechanisms guide microglia-neuron interactions in stress. Evidence indicates that neuronal activity-dependent purinergic signaling directs microglial
processes and synaptic engagement via P2Y12, a purinergic receptor exclusively expressed by microglia in the brain. Stress alters excitatory neurotransmission in the PFC, thus we aimed to
determine if P2Y12 signaling promotes functional changes in microglia in chronic stress. Here we used genetic ablation of P2Y12 (_P2ry12__–/–_) or pharmacological blockade (clopidogrel,
ticagrelor) to examine the role of purinergic signaling in stress-induced microglia-neuron interaction. Multiple behavioral, physiological, and cytometric endpoints were analyzed. Deletion
of P2Y12 led to a number of fundamental alterations in the PFC, including the heightened microglial number and increased dendritic spine density. Flow cytometry revealed that microglia in
_P2ry12–/–_ mice had shifts in surface levels of CX3CR1, CSF1R, and CD11b, suggesting changes in synaptic engagement and phagocytosis in the PFC. In line with this, pharmacological blockade
of P2Y12 prevented CUS-induced increases in the proportion of microglia with neuronal inclusions, limited dendritic spine loss in the PFC, and attenuated alterations in stress coping
behavior and working memory function. Overall, these findings indicate that microglial P2Y12 is a critical mediator of stress-induced synapse loss in the PFC and subsequent behavioral
deficits. You have full access to this article via your institution. Download PDF SIMILAR CONTENT BEING VIEWED BY OTHERS NUCLEAR GAPDH IN CORTICAL MICROGLIA MEDIATES CELLULAR STRESS-INDUCED
COGNITIVE INFLEXIBILITY Article 13 April 2024 THE RAP1 SMALL GTPASE IS A CRITICAL MEDIATOR OF THE EFFECTS OF STRESS ON PREFRONTAL CORTICAL DYSFUNCTION Article 10 July 2020 MICROGLIAL TYPE I
INTERFERON SIGNALING MEDIATES CHRONIC STRESS-INDUCED SYNAPSE LOSS AND SOCIAL BEHAVIOR DEFICITS Article 02 August 2024 INTRODUCTION Alterations in synaptic plasticity are thought to underlie
numerous stress-linked psychiatric disorders, including major depressive disorder (MDD) [1, 2]. For instance, patients suffering from MDD have fewer synapses in the prefrontal cortex (PFC)
and this correlates with MDD severity and working memory dysfunction [3]. Clinical and preclinical findings suggest that microglia, the brain’s resident macrophages, may contribute to
synaptic alterations in the PFC and MDD-associated symptoms. In fact, chronic stress has been shown to induce microglia-mediated remodeling of synapses in the medial PFC (mPFC) of mice and
subsequent deficits in PFC-mediated behaviors, including working memory function and cognitive flexibility [4, 5]. Despite these findings, relatively little is known regarding the pathways
that drive microglia-neuron interactions in stress and microglial interventions in stress-linked disorders remain elusive. Studies indicate that initial stress exposure increases pyramidal
neuron activity and glutamatergic signaling, and disturbs neuronal homeostasis in the PFC [6, 7]. Microglia respond dynamically to changes in neuronal activity by detecting the extrasynaptic
release of purines, including adenosine-diphosphate and triphosphate (ADP and ATP) [8, 9]. Purine release by neurons attracts microglial processes via P2Y12, a purinergic receptor
exclusively expressed by microglia in the brain [10,11,12]. These activity-dependent interactions have functional consequences as mice with genetic deletion of P2Y12 (_P2ry12__–/–_) show
impaired plasticity in the visual cortex following monocular deprivation during neurodevelopment and this was mediated, in part, by reduced uptake of synaptic material by microglia [10].
Thus, it is plausible that P2Y12 plays an important role in guiding stress-induced changes in microglia-mediated synaptic remodeling in the mPFC and associated behavioral consequences. To
investigate this, we disrupted P2Y12 signaling during chronic stress using either genetic or pharmacological tactics, with analyses addressing microglia-neuron interactions in the mPFC,
synapse density in this region, stress physiology, and cognitive-behavioral function. METHODS AND MATERIALS See online Supplementary Methods and Materials for full experimental details and
product/reagent information. ANIMALS Transgenic _P2ry12–/–_ and Thy1-GFP(M) mice were obtained from in-house breeders backcrossed to C57BL/6 J mice (6–8 weeks old). Thy1-GFP(M)/P2Y12
transgenic mice were generated by crossing lines. Pharmacological experiments were performed in both wild-type C57BL/6 J mice (behavior and flow cytometry) and Thy1-GFP(M) mice (histological
analyses). Primary studies used male mice as microglia-mediated neuronal remodeling in the PFC occurs in stressed male- but not female- mice [5, 13]. Behavioral and physiological outcomes
were examined in _P2ry12__–/–_ females. Animal procedures were approved by the University of Cincinnati Institutional Animal Care and Use Committees (IACUC) and were in accordance with
guidelines established by the National Institutes of Health. CHRONIC UNPREDICTABLE STRESS (CUS) CUS was performed as previously described [5, 14]. In brief, mice were exposed to two random
intermittent stressors per day for 14 days, including: cage rotation, isolation, restraint, radio noise, food or water deprivation, light on overnight, light off during day, rat odor,
stroboscope overnight, crowding, wet bedding, no bedding, or tilted cage. To validate this manipulation, body weight was measured at the start and end of CUS, with percent weight gain
calculated. Additional physiological analyses were conducted in _P2ry12__–/–_ mice. DRUG ADMINISTRATION Mice received either clopidogrel (50 mg/kg) or ticagrelor (10 mg/kg) via daily
intraperitoneal (i.p.) injection [10]. The bioactive metabolite of clopidogrel can cross the blood-brain barrier, where it irreversibly binds to microglial P2Y12 [10, 15]. In contrast, the
P2Y12 antagonist ticagrelor is not blood-brain barrier permeant, with studies showing no effect of peripheral ticagrelor treatment on microglial motility or injury responsivity [10]. Control
mice received equal volume injections of vehicle. BEHAVIOR AND COGNITIVE TESTS Open field test (OFT), forced swim test (FST) and temporal object recognition testing (TOR) were conducted as
previously described [5, 14, 16]. The mPFC contributes to both stress coping behavior in the FST and discrimination in the TOR, making these behavioral assays particularly relevant to the
neurobiological outcomes examined in this study [17, 18]. The open field test was used to assess animal mobility in a novel context and thigmotaxis. For the OFT, mobility and the total time
spent in the center zone of the open field were assessed. Time spent immobile (2–6 min phase) in the FST was measured. For the TOR, the time spent exploring objects was measured and a
discrimination index was calculated. PERCOLL GRADIENT ENRICHMENT OF MICROGLIA AND FLOW CYTOMETRY Dissected frontal cortex was homogenized and cells were enriched using Percoll. Cells were
incubated with conjugated antibodies (FITC-CX3CR1 or AlexaFluor488-CD115; PE-P2Y12 or PE-CD68; PerCp-Cy5-CD11b; PE(594)-CD45). An average of 25,434 ± 971 microglia were examined per sample
based on CD11b/CD45lo expression using a BioRad S3e four-color cytometer/cell sorter (Hercules, CA). Data were analyzed using FlowJo software (Ashland, OR). IMMUNOHISTOLOGY Mice were
perfused approximately 4 h after the final stressor or 2 h after behavioral testing. Brains were frozen and sectioned (40 µm). Free-floating sections containing the PFC were washed, blocked,
and incubated with primary antibody: rabbit anti-IBA1, rabbit anti-P2Y12, rat anti-CD68, or rabbit anti-FOSB. Sections were washed and incubated with conjugated secondary antibody (Alexa
Fluor 488, 546, and 647). QUANTITATIVE IMMUNOFLUORESCENCE Confocal images were captured from adjacent brain sections in both hemispheres of the mPFC (3-4 sections/sample spanning the
rostral-caudal axis, 2.10–1.34 mm Bregma). FosB immunolabeled tissue sections were imaged using a 10× objective. To quantify FosB+ cells, bright immunolabled cell bodies were thresholded and
then counted using the ImageJ (NIH) Analyze Particles function. FosB+ cell density was calculated as cells per mm2. For analysis of IBA1 + material and P2Y12 intensity, tissue sections were
imaged using a 20×. Individual IBA1 + and P2Y12 + cell counts were obtained by hand and cell density was calculated (cells per mm2). For microglial area, images were thresholded, total area
was recorded (µm2), and area per microglial cell was calculated (µm2/cell). To assess microglial clustering, the distance between each individual microglia and their closest neighboring
microglia was measured using the Nearest Neighbor Distance plugin. For analysis of microglial P2Y12 expression, images were thesholded, fluorescence intensity was measured (integrated
density, A.U.), and P2Y12 expression per microglial cell was calculated as fluorescence intensity/total number of IBA1 + cells per image (A.U., relative to control). In Thy1-GFP(M) mice,
layer I of the mPFC was identified in multiple adjacent sections and apical dendritic segments were imaged using either a 40x or 60x objective. Given the expression profile of GFP in this
transgenic line, apical segments in layer I most likely originate from pyramidal cells in layer V and VI of the mPFC [19]. Dendritic segments were traced and measured in NeuronStudio with
spines counted by hand (6–8 segments/sample). To quantify GFP + inclusions (total number and volume), confocal images were obtained in multiple adjacent sections and microglia with complete
morphological profiles were identified. Images were collected with sufficient resolution to detect synaptic inclusions (average inclusion diameter: 0.25 µm) [20]. Inclusions were identified
both within microglial processes and in microglial somata. In addition to GFP + inclusions, the total volume of microglial CD68 was measured in these images, with data expressed as CD68
volume (µm3)/total number of IBA1 + cells (µm3/IBA1 + cell). Of 629 analyzed GFP + inclusions, 619 (98.4%) colocalized with immunolabeled CD68. To examine autofluorescent lysosomal
inclusions, microglia were visualized in _P2ry12__–/–_ mice lacking the Thy1-GFP(M) transgene with images collected as described above. Laser settings were maintained across studies.
Autofluorescent inclusions were imaged at 488 nm excitation with an emission spectra of 510–574 nm. STATISTICAL ANALYSIS Data were analyzed using GraphPad Prism 8.1.2 (La Jolla, California).
Global main effects and interactions were determined using two-way ANOVA (all factors between-subjects; stress×genotype, stress×drug treatment). Following a significant ANOVA finding (main
effect or interaction), a series of hypothesis-informed, planned comparisons were conducted using Sidak’s multiple comparisons test [21,22,23,24]. For _P2ry12__–/–_ studies, the following
comparisons were made: wild-type control vs wild-type CUS, wild-type control vs _P2ry12__–/–_ control, wild-type control vs _P2ry12__–/–_ CUS, and _P2ry12__–/–_ control vs _P2ry12__–/–_ CUS.
For pharmacological experiments comparing clopidogrel and ticagrelor, animals treated with either 0.9% saline or DMSO:saline did not differ across any metrics and were thus collapsed into
one vehicle-treated group for analysis. For these studies, the following planned comparisons were made: vehicle control vs vehicle CUS, vehicle control vs clopidogrel control, vehicle
control vs ticagrelor control, vehicle control vs clopidogrel CUS, vehicle control vs ticagrelor CUS, clopidogrel control vs clopidogrel CUS, and ticagrelor control vs ticagrelor CUS.
Pearson correlation coefficients were computed and analyzed for select variables. The number of animals examined in each analysis is noted in Table S1, with complete omnibus statistics and
pairwise comparisons (including confidence intervals) reported in Table S2 and Table S3, respectively. RESULTS GENETIC LOSS OF P2Y12 ATTENUATES STRESS-INDUCED BEHAVIORAL CONSEQUENCES AND
INDUCES SIGNIFICANT ALTERATIONS IN FRONTAL CORTEX MICROGLIA To determine the role of P2Y12 in stress-induced microglia responses, wild-type and _P2ry12__–/–_ mice were exposed to 14 days of
CUS and then physiological, behavioral, and molecular outcomes were assessed (Fig. 1A). Both unstressed and CUS-exposed mice moved a comparable distance- and spent a similar amount of time
in the center zone in the OFT (Fig. 1B, C). Loss of P2Y12 caused a modest reduction in time spent in the center zone in the OFT (F(1,39) = 6.898, _p_ = 0.012), however, no pairwise
differences were detected following planned comparisons. Consistent with prior studies, CUS increased immobility in the FST (F(1,40) = 7.784, _p_ = 0.008), with pairwise comparisons
indicating greater immobility in wild-type (_p_ = 0.049), but not _P2ry12__–/–_ mice (Fig. 1D). CUS also reduced object discrimination in the TOR (F(1,40) = 23.83, _p_ < 0.0001), with
planned comparisons indicating significant differences in wild-type mice only (_p_ < 0.0001; Fig. 1E). The total amount of time exploring objects in the TOR was unaffected by stress or
genotype (Fig. 1F). Following behavioral testing, we examined whether P2Y12 ablation disrupts physiological stress responsivity. Stressed animals were subjected to a 1 h acute restraint
challenge followed by analysis of plasma corticosterone, body weight, and organ weight. Acute restraint elevated plasma corticosterone levels in both wild-type and _P2ry12__–/–_ mice
(F(1,38) = 57.34, _p_ < 0.0001; Fig. 1G). Additional analyses show that CUS reduced weight gain (Fig. 1H) and caused adrenal hypertrophy in mice regardless of genotype (Fig. 1I); spleen
weight was unaffected by CUS (Fig. 1J). Parallel studies in female mice found no effects of stress or genotype on behavior, despite CUS-induced alterations in weight gain and
stress-sensitive organ weight (Fig. S1A–G). Together, these findings indicate that genetic loss of _P2ry12_ in male mice attenuated behavioral responses to stress – despite a pronounced
physiological response. In subsequent experiments, surface markers were characterized on frontal cortex microglia using flow cytometry. Analyses of cell surface expression confirmed the
absence of P2Y12 in _P2ry12__–/–_ mice (F(1,28) = 295.0, _p_ < 0.0001; Fig. 1K, L). Further panels revealed that frontal cortex microglia from _P2ry12__–/–_ mice are phenotypically
different from wild-type microglia. In particular, frontal cortex microglia in _P2ry12__–/–_ mice had increased protein levels of CSF1R (F(1,28) = 7.719, _p_ = 0.001), CD11b (F(1,28) =
137.6, _p_ < 0.0001), and CD68 (F(1,28) = 102.2, _p_ < 0.0001), as well as decreased CX3CR1 expression (F(1,28) = 244.4, _p_ < 0.0001). Moreover, microglia from _P2ry12__–/–_ mice
showed higher side scatter (SSC), suggesting an increase in intracellular complexity (F(1,28) = 7.905, _p_ = 0.009). In addition, CUS increased microglial CSF1R, with pairwise comparisons
indicating that this increase was only significant in _P2ry12__–/–_ mice (_p_ < 0.001). Alongside CSF1R, exposure to CUS increased SSC (_p_ = 0.008) in _P2ry12__–/–_ mice. These findings
indicate that loss of P2Y12 leads to significant alterations in microglial phenotype and responsivity to CUS. LOSS OF P2Y12 CAUSES DYSREGULATION OF MICROGLIAL PHAGOCYTOSIS IN THE MEDIAL
PREFRONTAL CORTEX AND PREVENTS MICROGLIA-MEDIATED DENDRITIC REMODELING IN CHRONIC STRESS To examine neuron-microglia interactions, _P2ry12__–/–_ and wild-type mice were crossed with
Thy1-GFP(M) mice. Following 14 days of CUS brains were processed for confocal microscopy (Fig. S2A). Similar to prior work, exposure to CUS increased the number of FosB+ cells/mm2 in the
mPFC regardless of genotype (F(1,26) = 46.54, _p_ < 0.0001; Fig. S2B, C). Interestingly, _P2ry12__–/–_ mice had increased microglial density in the mPFC (F(1,26) = 83.95, _p_ < 0.0001;
Fig. S2B, D), contributing to a decrease in the distance between neighboring microglia (F(1,26) = 85.55, _p_ < 0.0001;Fig. S2E). Loss of P2Y12 had little effect on basal levels of IBA1 +
area per microglia in the mPFC (Fig. S2F). However, CUS increased microglial area in the mPFC in wild-type, but not _P2ry12__–/–_ mice (F(1,26) = 11.27, _p_ = 0.002). Assessment of
microglia-neuron interaction in layer I of the mPFC (Fig. 2A, B) revealed a CUS-induced increase in the proportion of microglia with GFP + inclusions in wild-type mice (F(1,25) = 80.05, _p_
< 0.0001). Surprisingly, we also observed high levels of GFP + inclusions in PFC microglia in both unstressed and stressed _P2ry12-/-_ mice. Control measures indicate a similar amount of
GFP + neuronal material in layer I of the mPFC across groups, regardless of genotype or CUS (Fig. S3A–C). Thus, it is unlikely that basal differences in the abundance of GFP + material
contributed to the increase in microglial inclusions detected here. To expand on this finding, we quantified the cellular location of these inclusions in microglia and determined the
proportion of GFP + inclusions exclusively in their soma, exclusively in their processes, and within both their soma and processes (Fig. 2B). These analyses showed that CUS reduced the
proportion of microglia with GFP + inclusions only in their soma (F(1,25) = 17.74, _p_ < 0.001), and increased the proportion of microglia with both somatic and process inclusions
(F(1,25) = 17.42, _p_ < 0.001) in wild-type, but not _P2ry12__–/–_ mice. The basal accumulation of GFP + inclusions in _P2ry12__–/–_ mice was primarily somatic, with very few process
inclusions detected (F(1,25) = 24.03, _p_ < 0.0001). Regardless of cellular localization, both stress and genotype had significant effects on the number of GFP + inclusions per phagocytic
microglia (F(1,25) = 4.275, _p_ = 0.049, Fig. 2C), and the volume of CD68 + material per microglia (F(1,25) = 13.82, _p_ = 0.001, Fig. 2D, E) in the mPFC. In line with these findings,
exposure to CUS reduced spine density on apical dendritic arbors in layer I of the mPFC in wild-type, but not _P2ry12__–/–_ mice (F(1,27) = 7.000, _p_ = 0.013, Fig. 2F). Prior studies
indicate that accumulation of lysosomes in the cell soma may present as autofluorescent material in microglia (e.g., lysosomal bodies containing lipid droplets, cholesterol crystals) [25].
To test this, PFC microglia with CD68 + lysosomes were visualized in wild-type and _P2ry12__–/–_ mice lacking the Thy1-GFP(M) transgene. In wild-type mice there were few autofluorescent CD68
+ lysosomes, however, autofluorescent material was observed in somatic lysosomes in _P2ry12__–/–_ mice (Fig. S4A, B). The mean intensity of these somatic accumulations was significantly
lower than that of inclusions observed in mice carrying the Thy1-GFP(M) transgene (Fig. S4C). These results indicate that GFP + inclusions in microglia are composed of neuronal material in
wild-type Thy1-GFP(M) mice. In contrast, it appears that GFP + inclusions in microglia from _P2ry12__–/–_ Thy1-GFP(M) animals are composed of both autofluorescent and neuronal material.
Altogether, P2Y12 appears to play a critical role in regulating microglial density and lysosome function in the mPFC regardless of stress. These data further suggest that P2Y12 is important
in guiding stress effects on microglial morphology and microglia-neuron interactions in this region. PHARMACOLOGICAL BLOCKADE OF MICROGLIAL P2Y12 ATTENUATES STRESS EFFECTS ON BEHAVIOR AND
SHIFTS MICROGLIAL PHENOTYPE IN FRONTAL CORTEX To complement previous experiments, P2Y12 was manipulated pharmacologically using either clopidogrel, a P2Y12 antagonist that can access the
brain, or ticagrelor, an antagonist which cannot cross the blood-brain barrier. Unstressed and CUS-exposed mice treated with either vehicle, clopidogrel, or ticagrelor were assessed in the
FST and TOR (Fig. 3A). CUS increased immobility in the FST (F(2,71) = 5.239, _p_ = 0.008) in vehicle- (_p_ = 0.012) and ticagrelor-treated mice (_p_ = 0.010), but not clopidogrel-treated
animals (Fig. 3B). Similar to the FST, CUS caused deficits in temporal object recognition (F(1,72) = 23.92, _p_ < 0.0001) which were only detected in vehicle- (_p_ < 0.0001) and
ticagrelor-treated mice (_p_ = 0.006; Fig. 3C). Drug treatment affected the total time spent exploring objects in the TOR (F(2,72) = 4.212, _p_ = 0.019), however, pairwise comparisons
uncovered no specific group level differences (Fig. 3D). Exposure to CUS reduced weight gain in both vehicle- and clopidogrel-treated animals (Table S2, S3, Fig. S5). Collectively, these
findings indicate that blocking P2Y12 on microglia (i.e., clopidogrel) but not peripheral cells (i.e. ticagrelor) attenuates stress effects on behavior. In a separate cohort, we analyzed the
phenotype of frontal cortex microglia using flow cytometry (Fig. 3E). Similar to genetic loss, treatment with clopidogrel reduced expression of P2Y12 (F(2,79) = 98.59, _p_ < 0.0001) and
CX3CR1 (F(2,62) = 30.39, _p_ < 0.0001). There was an overall effect of drug treatment on microglial CD11b (F(2,83) = 3.390, _p_ = 0.038); however, no significant differences emerged with
pairwise comparisons. Increased levels of microglial CSF1R were observed in mice treated with clopidogrel (F(2,60) = 5.964, _p_ = 0.004) and those exposed to CUS (F(1,60) = 10.66, _p_ =
0.002). Planned comparisons showed that CUS increased microglial CSF1R in both vehicle- (_p_ = 0.044) and ticagrelor-treated mice (_p_ = 0.040), but not clopidogrel-treated mice. Neither
drug treatment nor CUS had an effect on microglial SSC. Thus, pharmacological blockade of P2Y12 caused changes in microglial phenotype that resembled genetic loss, while also preventing
stress effects on CSF1R expression in frontal cortex microglia. INHIBITING MICROGLIAL P2Y12 BLOCKS STRESS-INDUCED PHAGOCYTOSIS OF NEURONAL ELEMENTS AND ASSOCIATED DENDRITIC SPINE LOSS IN THE
MPFC We next analyzed neuronal function, microglial phenotype, and microglia-neuron interaction in the mPFC using confocal microscopy (Fig. 4A). CUS increased the number of FosB+ cells/mm2
in the mPFC in both vehicle- and clopidogrel- treated animals (F(1,26) = 24.18, _p_ < 0.0001; Fig. 4B, C). Neither CUS nor treatment with clopidogrel affected the number of IBA1 +
cells/mm2, P2Y12 + cells/mm2, or microglial nearest neighbor distance in the mPFC (Fig. 4D, Table S2). However, CUS increased microglial morphological area, and this was blocked by treatment
with clopidogrel (F(1,26) = 13.59, _p_ = 0.001; Fig. 4E). Similar to our flow cytometry findings, treatment with clopidogrel reduced microglial P2Y12 intensity in the PFC (F(1,26) = 22.59,
_p_ < 0.0001; Fig. 4F). Exposure to CUS increased the proportion of microglia with GFP + inclusions in the mPFC in wild-type, but not clopidogrel-treated mice (F(1,22) = 13.52, _p_ =
0.001; Fig. 5A, B). Analysis of inclusion location revealed a CUS-induced reduction in the proportion of microglia with soma-only inclusions (F(1,22) = 4.343, _p_ = 0.049) and an increase in
the proportion of microglia with both somatic and process inclusions (F(1,22) = 8.018, _p_ < 0.001) in vehicle-treated animals (Fig. 5B). Further analyses indicate that treatment with
clopidogrel prevented CUS-induced increases in the number of GFP + inclusions (F(1,22) = 5.606, _p_ = 0.027; Fig. 5C), the average volume of GFP + inclusions per phagocytic microglia
(F(1,22) = 15.94, _p_ = 0.001; Fig. 5D), and the accumulation of CD68 + lysosomes in microglia (F(1,26) = 12.55, _p_ = 0.002; Fig. 5E). In line with these data, blocking microglial P2Y12
with clopidogrel prevented spine loss on apical dendrites in the mPFC of CUS-exposed mice (F(1,21) = 12.98, _p_ = 0.002; Fig. 5F). Correlational analyses indicate a significant association
between FosB induction in the mPFC and an increased proportion of microglia with neuronal inclusions (_r_ = 0.896, _p_ < 0.0001) in vehicle-, but not clopidogrel-treated animals (Fig.
5G). This increase in the proportion of microglia with neuronal inclusions was strongly associated with reductions in dendritic spine density in vehicle-treated mice (_r_ = −0.845, _p_ <
0.0001). Together, these findings suggest that CUS increases neuronal activity in the mPFC, and this engages microglia and subsequently triggers microglia-mediated dendritic remodeling
through a P2Y12-dependent pathway. DISCUSSION Various stress-linked psychiatric disorders, including MDD, are marked by dendritic atrophy and synapse loss in the PFC. Recent preclinical
studies suggest that microglia contribute to stress-induced changes in neuronal structure and associated behavioral consequences [4, 5, 14, 26], however, the pathways that direct
microglia-neuron interactions in this context remain unclear. Our primary findings indicate that P2Y12 signaling is required for stress-induced microglial phagocytosis of neuronal elements
and mediates spine loss on pyramidal neurons in the PFC, which underlies deficits in working memory and stress-coping behavior. Our data also demonstrate that P2Y12 is essential for
establishing microglial phenotype in the PFC, regardless of stress. P2Y12 IS A CRITICAL REGULATOR OF MICROGLIAL PHENOTYPE AND FUNCTION Studies have identified P2Y12 as a critical receptor in
the microglial ‘sensome’ [27], guiding microglial surveillance and physical microglia-synapse contact [8, 10, 28, 29]. In line with these findings, our data show that P2Y12 broadly
regulates microglial phenotype and function in the frontal cortex. Genetic loss or pharmacological blockade of P2Y12 shifted the homeostatic phenotype of microglia with increased surface
protein levels of CSF1R and CD11b, and decreased expression of CX3CR1. This is relevant because CSF1R and CD11b have been shown to drive microglia-neuron interactions and synaptic remodeling
[5, 30], whereas CX3CR1-signaling can either promote- or suppress- aspects of microglial function [31, 32]. These phenotypic alterations are likely a compensatory mechanism through which
microglia attempt to survey and modulate synaptic structures with deficient P2Y12 signaling. Another important finding is that genetic loss, but not pharmacological blockade, of P2Y12
increased the density and morphology of microglia in the PFC of unstressed adult mice. This aligns with reports showing that P2Y12 guides rearrangement of the broad microglial landscape and
microglial translocation [28]. In addition, microglia in unstressed _P2ry12__–/–_ mice accumulated large somatic CD68 + inclusions composed of both autofluorescent and neuronal material.
These inclusions may have contributed to an increase in microglial SSC in _P2ry12__–/–_ mice, and suggest impairments in microglial lysosome regulation and increased levels of phagocytosis
and/or autophagy [25]. Interestingly, this increase in microglial phagocytosis was not associated with a loss of dendritic spines in _P2ry12–/–_ mice. Other studies have established that
ablation of P2Y12 reduces microglial phagocytosis of GluR1+ synapses and disrupts experience-dependent synaptic plasticity in the developing visual cortex [10]. Considering this, alongside
the discordant microglial phenotype observed (i.e., heightened levels of CSF1R, CD11b, CD68), it is possible that microglia are remodeling other dendritic structures not examined in this
study or that microglia are contributing to a greater turnover of dendritic material in mice lacking P2Y12. Future studies will be needed to determine the composition of microglial
inclusions and mechanisms that lead to their accumulation in _P2ry12__–/–_ mice. Despite these robust neurobiological effects, no behavioral alterations were detected in mice lacking P2Y12.
This is consistent with a recent report showing only modest shifts in cognition and behavior in _P2ry12__–/–_ mice [33]. STRESS-INDUCED MICROGLIA-MEDIATED DENDRITIC REMODELING, SYNAPSE LOSS,
AND BEHAVIORAL ADAPTATIONS IN STRESS ARE DEPENDENT ON P2Y12 SIGNALING Stress exposure increases neuronal activity, alters excitatory neurotransmission, and purine metabolism in the PFC [34,
35]. Prior work indicates that elevated neuronal activity ‘attracts’ microglial processes via extracellular purines acting on microglial P2Y12 [9]. This purinergic signaling pathway
promotes microglia-neuron interactions, which shape neuroplasticity and circuit-level function in various contexts [10, 12, 29, 36, 37]. In this study, CUS increased expression of the
neuronal activation marker FosB in the PFC and disrupted both stress-coping behavior and working memory. Administration of the brain permeant P2Y12 antagonist clopidogrel attenuated these
CUS-induced deficits, whereas treatment with ticagrelor, which cannot cross the blood-brain barrier, did not prevent behavioral consequences [10, 15]. Thus, blocking P2Y12 on microglia,
specifically, reduces stress effects on behavioral and cognitive outcomes mediated by the PFC. Chronic stress increases microglia-neuron interaction and microglial process-mediated synapse
elimination in the mPFC [4, 38]. Consistent with these findings, we show that CUS causes microglia in the mPFC to accumulate neuronal elements in both their processes and cell body,
suggesting process-mediated actions (e.g., synaptic pruning) and somatic debris compartmentalization (e.g., in lysosomes) [4, 5, 31, 39]. This accumulation of neuronal material within
microglia was strongly associated with reductions in dendritic spine density, further implicating microglia in CUS-induced neuronal remodeling [4]. Genetic knockout or pharmacological
blockade of microglial P2Y12 prevented microglia-mediated neuronal remodeling and attenuated synapse loss in the mPFC. Considering this, alongside our prior data connecting neuronal activity
and microglial function [26], it is plausible that CUS-induced activation of excitatory neurons in the mPFC engages P2Y12 signaling in microglia, which increases the frequency of
microglia-synapse interactions and subsequent microglia-mediated dendritic remodeling (Fig. S6). This would be in line with other studies showing that P2Y12 is necessary for microglia to
respond to fear- and seizure-associated activity in the hippocampus [29, 40], and that microglial P2Y12 can regulate experience-dependent synaptic plasticity [10]. It is important to note
that the stress response is dynamic and likely engages other microglial pathways that are capable of modulating neuronal function [41]. Interestingly, a recent study showed that neuronal
hyperactivity triggered a signaling cascade that involved P2Y12 and resulted in microglia providing negative regulatory feedback through release of the inhibitory molecule adenosine [36]. In
this context, it is possible that P2Y12 signaling drives varied microglia functions across the duration of CUS. Additional studies will be needed to directly examine these temporal
dynamics. Exposure to stress differentially alters microglia in the mPFC in male and female mice and rats. For instance, chronic stress increases microglial morphological area in males yet
has little effect on- or decreases microglial area in female rodents [13, 42, 43]. Moreover, microglial actions have been associated with stress-induced synapse loss in the mPFC and
subsequent deficits in behavior in male, but not female, mice [13]. In this study, genetic ablation of P2Y12 had no effect on physiological stress responsivity, stress coping behavior, or
temporal object recognition in female mice exposed to CUS. These findings, in concert with other reports, suggest a sex-specific role for microglia in regulating neuroplasticity and behavior
in stress [44,45,46,47,48]. Notably, post-mortem transcriptomic studies indicate similar sex-dependent microglial and synapse-associated patterns in the dorsolateral PFC (dlPFC) in MDD; men
show increased expression of microglial genes and decreased synapse-associated transcripts, whereas women show the opposite [49]. Future experiments characterizing sex differences in
microglia and mechanisms mediating these effects are warranted [50]. The findings presented here may be translationally relevant, as recent studies show that microglia isolated from
individuals diagnosed with MDD have heightened expression of ‘sensome’ markers, including P2Y12 and TMEM119 [51, 52]. Intriguingly, these same studies found no differential expression of
inflammation-associated molecules, suggesting that MDD is not marked by microglial ‘activation’ or neuroinflammation, but rather a more nuanced shift in microglial phenotype. This
parainflammatory phenotype is observed in several models of chronic stress [53]. Given our data, it is interesting to speculate that microglial P2Y12 may play an important role in regulating
synapse loss in MDD. CONCLUSION Collectively, our findings demonstrate a role for microglial P2Y12 in guiding stress effects on prefrontal structure, cognition, and behavior. These data may
be clinically pertinent, as both prefrontal synapse loss and altered levels of microglial P2Y12 have been found in individuals diagnosed with MDD. This work is significant as it provides
insight into pathways that direct microglia-neuron interactions, and how these interactions modulate the synaptic structure and behavioral responses in stress-linked psychopathology.
REFERENCES * Thompson SM, Kallarackal AJ, Kvarta MD, Van Dyke AM, LeGates TA, Cai X. An excitatory synapse hypothesis of depression. Trends Neurosci. 2015;38:279–94. CAS PubMed PubMed
Central Google Scholar * Duman RS, Aghajanian GK, Sanacora G, Krystal JH. Synaptic plasticity and depression: New insights from stress and rapid-acting antidepressants. Nat Med.
2016;22:238–49. * Holmes SE, Scheinost D, Finnema SJ, Naganawa M, Davis MT, DellaGioia N, et al. Lower synaptic density is associated with depression severity and network alterations. Nature
Commun. 2019;10:1529. Google Scholar * Liu T, Lu J, Lukasiewicz K, Pan B, Zuo Y. Stress induces microglia-associated synaptic circuit alterations in the dorsomedial prefrontal cortex.
Neurobiol Stress. 2021:100342. * Wohleb ES, Terwilliger R, Duman CH, Duman RS. Stress-induced neuronal CSF1 provokes microglia-mediated neuronal remodeling and depressive-like behavior. Biol
Psychiatry. 2018;83:38–49. CAS PubMed Google Scholar * Yuen EY, Liu W, Karatsoreos IN, Feng J, McEwen BS, Yan Z. Acute stress enhances glutamatergic transmission in prefrontal cortex and
facilitates working memory. Proc Natl Acad Sci USA. 2009;106:14075–9. CAS PubMed PubMed Central Google Scholar * Miner LAH, Jedema HP, Moore FW, Blakely RD, Grace AA, Sesack SR. Chronic
stress increases the plasmalemmal distribution of the norepinephrine transporter and the coexpression of tyrosine hydroxylase in norepinephrine axons in the prefrontal cortex. J Neurosci.
2006;26:1571–8. CAS PubMed PubMed Central Google Scholar * Haynes SE, Hollopeter G, Yang G, Kurpius D, Dailey ME, Gan W-B, et al. The P2Y12 receptor regulates microglial activation by
extracellular nucleotides. Nat Neurosci. 2006;9:1512. CAS PubMed Google Scholar * Dissing-Olesen L, LeDue JM, Rungta RL, Hefendehl JK, Choi HB, MacVicar BA. Activation of neuronal NMDA
receptors triggers transient ATP-mediated microglial process outgrowth. J Neurosci. 2014;34:10511. PubMed PubMed Central Google Scholar * Sipe GO, Lowery RL, Tremblay M, Kelly EA,
Lamantia CE, Majewska AK. Microglial P2Y12 is necessary for synaptic plasticity in mouse visual cortex. Nature. Communications. 2016;7:1–15. Google Scholar * Butovsky O, Jedrychowski MP,
Moore CS, Cialic R, Lanser AJ, Gabriely G, et al. Identification of a unique TGF-β–dependent molecular and functional signature in microglia. Nat Neurosci. 2013;17:131–43. PubMed PubMed
Central Google Scholar * Eyo UB, Peng J, Swiatkowski P, Mukherjee A, Bispo A, Wu L-JJ. Neuronal hyperactivity recruits microglial processes via neuronal NMDA receptors and microglial P2Y12
receptors after status epilepticus. J Neurosci. 2014;34:10528–40. PubMed PubMed Central Google Scholar * Woodburn SC, Bollinger JL, Wohleb ES. Synaptic and behavioral effects of chronic
stress are linked to dynamic and sex-specific changes in microglia function and astrocyte dystrophy. Neurobiol Stress. 2021;14:100312. * Horchar MJ, Wohleb ES. Glucocorticoid receptor
antagonism prevents microglia-mediated neuronal remodeling and behavioral despair following chronic unpredictable stress. Brain, Behavior, Immunity. 2019. 2019.
https://doi.org/10.1016/j.bbi.2019.06.030. * Tozaki-Saitoh H, Tsuda M, Miyata H, Ueda K, Kohsaka S, Inoue K. P2Y12 receptors in spinal microglia are required for neuropathic pain after
peripheral nerve injury. J Neurosci. 2008;28:4949–56. CAS PubMed PubMed Central Google Scholar * Yuen EY, Wei J, Liu W, Zhong P, Li X, Yan Z. Repeated stress causes cognitive impairment
by suppressing glutamate receptor expression and function in prefrontal cortex. Neuron. 2012;73:962–77. CAS PubMed PubMed Central Google Scholar * Warden MR, Selimbeyoglu A, Mirzabekov
JJ, Lo M, Thompson KR, Kim SY, et al. A prefrontal cortex–brainstem neuronal projection that controls response to behavioural challenge. Nature. 2012;492:428–32. 7429. 2012;492 CAS PubMed
PubMed Central Google Scholar * Barker GRI, Bird F, Alexander V, Warburton EC. Recognition memory for objects, place, and temporal order: A disconnection analysis of the role of the medial
prefrontal cortex and perirhinal cortex. J Neurosci. 2007. 2007. https://doi.org/10.1523/jneurosci.5289-06.2007. * Porrero C, Rubio-Garrido P, Avendaño C, Clascá F. Mapping of fluorescent
protein-expressing neurons and axon pathways in adult and developing Thy1-eYFP-H transgenic mice. Brain Res. 2010;1345:59–72. CAS PubMed Google Scholar * Weinhard L, di Bartolomei G,
Bolasco G, Machado P, Schieber NL, Neniskyte U, et al. Microglia remodel synapses by presynaptic trogocytosis and spine head filopodia induction. Nat Commun. 2018;9:1228. PubMed PubMed
Central Google Scholar * Wei J, Carroll RJ, Harden KK, Wu G. Comparisons of treatment means when factors do not interact in two-factorial studies. Amino Acids. 2012;42:2031–5. CAS PubMed
Google Scholar * Weissgerber TL, Garcia-Valencia O, Garovic VD, Milic NM, Winham SJ. Why we need to report more than ‘Data were Analyzed by t-tests or ANOVA’. ELife. 2018;7:e36163. PubMed
PubMed Central Google Scholar * Ruxton GD, Beauchamp G. Time for some a priori thinking about post hoc testing. Behav Ecol. 2008;19:690–3. Google Scholar * Alger BE. Neuroscience needs
to test both statistical and scientific hypotheses. J Neurosci. 2022;42:8432–8. CAS PubMed PubMed Central Google Scholar * Burns JC, Cotleur B, Walther DM, Bajrami B, Rubino SJ, Wei R,
et al. Differential accumulation of storage bodies with aging defines discrete subsets of microglia in the healthy brain. ELife. 2020;9:1–71. Google Scholar * Bollinger JL, Horchar MJ,
Wohleb ES. Diazepam limits microglia-mediated neuronal remodeling in the prefrontal cortex and associated behavioral consequences following chronic unpredictable stress.
Neuropsychopharmacology. 2020;45:1766–76. CAS PubMed PubMed Central Google Scholar * Hickman SE, Kingery ND, Ohsumi TK, Borowsky ML, Wang LC, Means TK, et al. The microglial sensome
revealed by direct RNA sequencing. Nat Neurosci. 2013;16:1896–905. CAS PubMed PubMed Central Google Scholar * Eyo UB, Mo M, Yi M-H, Murugan M, Liu J, Yarlagadda R, et al.
P2Y12R-dependent translocation mechanisms gate the changing microglial landscape. Cell Rep. 2018;23:959–66. CAS PubMed PubMed Central Google Scholar * Mo M, Eyo UB, Xie M, Peng J, Bosco
DB, Umpierre AD, et al. Microglial P2Y12 receptor regulates seizure-induced neurogenesis and immature neuronal projections. J. Neurosci. 2019;39:9453–64. CAS PubMed PubMed Central Google
Scholar * Schafer DP, Lehrman EK, Kautzman AG, Koyama R, Mardinly AR, Yamasaki R, et al. Microglia sculpt postnatal neural circuits in an activity and complement-dependent manner. Neuron.
2012;74:691–705. CAS PubMed PubMed Central Google Scholar * Paolicelli RC, Bolasco G, Pagani F, Maggi L, Scianni M, Panzanelli P, et al. Synaptic pruning by microglia is necessary for
normal brain development. Science. 2011;333:1456–8. CAS PubMed Google Scholar * Cardona AE, Pioro EP, Sasse ME, Kostenko V, Cardona SM, Dijkstra IM, et al. Control of microglial
neurotoxicity by the fractalkine receptor. Nat Neurosci. 2006;9:917–24. CAS PubMed Google Scholar * Lowery RL, Mendes MS, Sanders BT, Murphy AJ, Whitelaw BS, Lamantia CE, et al. Loss of
P2Y12 has behavioral effects in the adult mouse. Int J Mol Sci. 2021;22:1868. CAS PubMed PubMed Central Google Scholar * Hamilton PJ, Chen EY, Tolstikov V, Peña CJ, Picone JA, Shah P, et
al. Chronic stress and antidepressant treatment alter purine metabolism and beta oxidation within mouse brain and serum. Sci Rep. 2020;10:18134. * Yue N, Huang H, Zhu X, Han Q, Wang Y, Li
B, et al. Activation of P2X7 receptor and NLRP3 inflammasome assembly in hippocampal glial cells mediates chronic stress-induced depressive-like behaviors. J Neuroinflammation. 2017;14:102.
PubMed PubMed Central Google Scholar * Badimon A, Strasburger HJ, Ayata P, Chen X, Nair A, Ikegami A, et al. Negative feedback control of neuronal activity by microglia. Nature.
2020;586:417–23. CAS PubMed PubMed Central Google Scholar * Lou N, Takano T, Pei Y, Xavier AL, Goldman SA, Nedergaard M. Purinergic receptor P2RY12-dependent microglial closure of the
injured blood–brain barrier. Proc Natl Acad Sci. 2016;113:1074–9. CAS PubMed PubMed Central Google Scholar * Wohleb ES, Terwilliger R, Duman CH, Duman RS. Stress-induced neuronal colony
stimulating factor 1 provokes microglia-mediated neuronal remodeling and depressive-like behavior. Biol Psychiatry. 2018;83:38–49. CAS PubMed Google Scholar * El Hajj H, Savage JC, Bisht
K, Parent M, Vallières L, Rivest S, et al. Ultrastructural evidence of microglial heterogeneity in Alzheimer’s disease amyloid pathology. J Neuroinflammation. 2019;16:87. PubMed PubMed
Central Google Scholar * Peng J, Liu Y, Umpierre AD, Xie M, Tian DS, Richardson JR, et al. Microglial P2Y12 receptor regulates ventral hippocampal CA1 neuronal excitability and innate fear
in mice. Mol Brain. 2019;12:71. * Yu T, Zhang X, Shi H, Tian J, Sun L, Hu X, et al. P2Y12 regulates microglia activation and excitatory synaptic transmission in spinal lamina II neurons
during neuropathic pain in rodents. Cell Death Dis. 2019;10:1–16. 10:3. 2019 Google Scholar * Bollinger JL, Burns CMB, Wellman CL. Differential effects of stress on microglial cell
activation in male and female medial prefrontal cortex. Brain Behav Immunity. 2016;52:88–97. CAS Google Scholar * Bollinger JL, Salinas I, Fender E, Sengelaub DR, Wellman CL. Gonadal
hormones differentially regulate sex-specific stress effects on glia in the medial prefrontal cortex. J Neuroendocrinol. 2019. 2019. https://doi.org/10.1111/jne.12762. * Gaspar R,
Soares-Cunha C, Domingues AV, Coimbra B, Baptista FI, Pinto L, et al. Resilience to stress and sex-specific remodeling of microglia and neuronal morphology in a rat model of anxiety and
anhedonia. Neurobiol Stress. 2021;14:100302. * Bolton JL, Short AK, Othy S, Kooiker CL, Shao M, Gunn BG, et al. Early stress-induced impaired microglial pruning of excitatory synapses on
immature CRH-expressing neurons provokes aberrant adult stress responses. Cell Rep. 2022;38:110600. CAS PubMed PubMed Central Google Scholar * Tsyglakova M, Huskey AM, Hurst EH, Telep
NM, Wilding MC, Babington ME, et al. Sex and region-specific effects of variable stress on microglia morphology. Brain, Behav Immunity - Health. 2021;18:100378. Google Scholar * Bollinger
JL, Collins KE, Patel R, Wellman CL. Behavioral stress alters corticolimbic microglia in a sex- and brain region-specific manner. PLOS ONE. 2017;12:e0187631. PubMed PubMed Central Google
Scholar * Caetano L, Pinheiro H, Patrício P, Mateus-Pinheiro A, Alves ND, Coimbra B, et al. Adenosine A2A receptor regulation of microglia morphological remodeling-gender bias in physiology
and in a model of chronic anxiety. Mol Psychiatry. 2017;22:1035–43. CAS PubMed Google Scholar * Seney ML, Huo Z, Cahill K, French L, Puralewski R, Zhang J, et al. Opposite Molecular
Signatures of Depression in Men and Women. Biol Psychiatry. 2018;84:18–27. CAS PubMed PubMed Central Google Scholar * Bollinger JL. Uncovering microglial pathways driving sex-specific
neurobiological effects in stress and depression. Brain, Behav Immunity - Health. 2021;16:100320. CAS Google Scholar * Böttcher C, Fernández-Zapata C, Snijders GJL, Schlickeiser S,
Sneeboer MAM, Kunkel D, et al. Single-cell mass cytometry of microglia in major depressive disorder reveals a non-inflammatory phenotype with increased homeostatic marker expression. Transl
Psychiatry. 2020;10:1–11. Google Scholar * Snijders GJLJ, Sneeboer MAM, Fernández-Andreu A, Udine E, Boks MP, Ormel PR, et al. Distinct non-inflammatory signature of microglia in
post-mortem brain tissue of patients with major depressive disorder. Mol Psychiatry. 2020; 26:3336–49. * Woodburn SC, Bollinger JL, Wohleb ES. The semantics of microglia activation:
neuroinflammation, homeostasis, and stress. J Neuroinflammation. 2021;18:1–16. 1. 2021;18 Google Scholar Download references ACKNOWLEDGEMENTS The authors would like to thank Dr. Ania
Majewska for kindly donating _P2ry12__–/–_ mice, and Dr. Grayson Sipe for advice regarding administration of clopidogrel and ticagrelor. The authors would also like to thank Dr. Lauren
Vollmer for feedback on this manuscript. Additional support was provided by the Statistics Consulting Center at the University of Cincinnati. This work was supported by the National
Institute of Mental Health (F32MH123051, JLB; R01MH123545, ESW) and the University of Cincinnati. AUTHOR INFORMATION AUTHORS AND AFFILIATIONS * Department of Pharmacology & Systems
Physiology, University of Cincinnati College of Medicine, Cincinnati, OH, USA Justin L. Bollinger, David T. Dadosky, James K. Flurer, Ivanka L. Rainer, Samuel C. Woodburn & Eric S.
Wohleb Authors * Justin L. Bollinger View author publications You can also search for this author inPubMed Google Scholar * David T. Dadosky View author publications You can also search for
this author inPubMed Google Scholar * James K. Flurer View author publications You can also search for this author inPubMed Google Scholar * Ivanka L. Rainer View author publications You can
also search for this author inPubMed Google Scholar * Samuel C. Woodburn View author publications You can also search for this author inPubMed Google Scholar * Eric S. Wohleb View author
publications You can also search for this author inPubMed Google Scholar CONTRIBUTIONS Study conception and design: JLB, ESW. Acquisition of data: JLB, DTD, JKF, ILR, SCW. Analysis and
interpretation of data: JLB, ESW. Drafting of paper: JLB, ESW. CORRESPONDING AUTHOR Correspondence to Eric S. Wohleb. ETHICS DECLARATIONS COMPETING INTERESTS The authors declare no competing
interests. ADDITIONAL INFORMATION PUBLISHER’S NOTE Springer Nature remains neutral with regard to jurisdictional claims in published maps and institutional affiliations. SUPPLEMENTARY
INFORMATION SUPPLEMENTAL METHODS, MATERIALS, AND FIGURES SUPPLEMENTAL TABLES RIGHTS AND PERMISSIONS Springer Nature or its licensor (e.g. a society or other partner) holds exclusive rights
to this article under a publishing agreement with the author(s) or other rightsholder(s); author self-archiving of the accepted manuscript version of this article is solely governed by the
terms of such publishing agreement and applicable law. Reprints and permissions ABOUT THIS ARTICLE CITE THIS ARTICLE Bollinger, J.L., Dadosky, D.T., Flurer, J.K. _et al._ Microglial P2Y12
mediates chronic stress-induced synapse loss in the prefrontal cortex and associated behavioral consequences. _Neuropsychopharmacol._ 48, 1347–1357 (2023).
https://doi.org/10.1038/s41386-022-01519-7 Download citation * Received: 09 August 2022 * Revised: 28 November 2022 * Accepted: 30 November 2022 * Published: 14 December 2022 * Issue Date:
August 2023 * DOI: https://doi.org/10.1038/s41386-022-01519-7 SHARE THIS ARTICLE Anyone you share the following link with will be able to read this content: Get shareable link Sorry, a
shareable link is not currently available for this article. Copy to clipboard Provided by the Springer Nature SharedIt content-sharing initiative