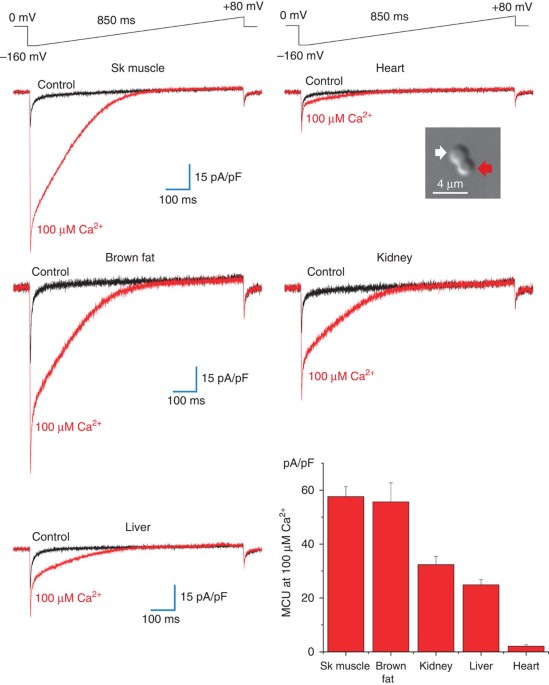
- Select a language for the TTS:
- UK English Female
- UK English Male
- US English Female
- US English Male
- Australian Female
- Australian Male
- Language selected: (auto detect) - EN
Play all audios:
ABSTRACT The mitochondrial calcium uniporter is a highly selective channel responsible for mitochondrial Ca2+ uptake. The mitochondrial calcium uniporter shapes cytosolic Ca2+ signals,
controls mitochondrial ATP production, and is involved in cell death. Here using direct patch-clamp recording from the inner mitochondrial membrane, we compare mitochondrial calcium
uniporter activity in mouse heart, skeletal muscle, liver, kidney and brown fat. Surprisingly, heart mitochondria show a dramatically lower mitochondrial calcium uniporter current density
than the other tissues studied. Similarly, in _Drosophila_ flight muscle, mitochondrial calcium uniporter activity is barely detectable compared with that in other fly tissues. As
mitochondria occupy up to 40% of the cell volume in highly metabolically active heart and flight muscle, low mitochondrial calcium uniporter activity is likely essential to avoid cytosolic
Ca2+ sink due to excessive mitochondrial Ca2+ uptake. Simultaneously, low mitochondrial calcium uniporter activity may also prevent mitochondrial Ca2+ overload in such active tissues exposed
to frequent cytosolic Ca2+ activity. You have full access to this article via your institution. Download PDF SIMILAR CONTENT BEING VIEWED BY OTHERS ELEMENTARY CALCIUM RELEASE EVENTS IN THE
SKELETAL MUSCLE CELLS OF THE HONEY BEE _APIS MELLIFERA_ Article Open access 18 August 2021 THE MITOCHONDRIAL PERMEABILITY TRANSITION PHENOMENON ELUCIDATED BY CRYO-EM REVEALS THE GENUINE
IMPACT OF CALCIUM OVERLOAD ON MITOCHONDRIAL STRUCTURE AND FUNCTION Article Open access 13 January 2021 THE ROLES OF MITOCHONDRIA IN GLOBAL AND LOCAL INTRACELLULAR CALCIUM SIGNALLING Article
27 January 2025 INTRODUCTION Mitochondrial Ca2+ uptake has long been recognized as instrumental in cellular physiological and pathophysiological conditions1,2,3. It controls the rate of
mitochondrial ATP production4,5, shapes cytosolic Ca2+ signals in heart and other tissues6,7,8,9,10, modulates the contraction-relaxation cycle of skeletal muscle11,12, regulates
immunological synapses13, and is involved in cell death1,14,15. Cytosolic Ca2+ influx into the mitochondrial matrix is mediated primarily by the mitochondrial calcium uniporter
(MCU)10,16,17. Patch-clamp studies in vesicles of the native inner mitochondrial membrane (IMM, mitoplasts) have demonstrated that the MCU is a highly selective Ca2+ channel with unique
properties18. Functional characterization of the MCU has been followed by a search for molecular candidates, and to date two molecules, MICU1 and MCU, have been shown to be important for the
MCU activity19,20,21,22. Mitochondrial Ca2+ uptake has been primarily studied indirectly using ion flux assays in suspensions of isolated mitochondria and by calcium imaging in intact
cells. These studies have led to the identification and characterization of the phenomenon of mitochondrial Ca2+ uptake _in vitro_17,23 and demonstrated that this uptake occurs under
physiological conditions in intact cells despite the low transport affinity of MCU for Ca2+ (refs 24,25). However, these methods do not measure MCU activity directly; thus, mitochondrial
potential (ΔΨm), matrix pH, and ion gradients across the IMM cannot be accurately controlled17,23,25. Therefore, it is not clear whether mitochondrial Ca2+ uptake mediated by the MCU is the
same among different tissues and whether any differences can influence the physiology of these tissues. Scarce evidence for the tissue heterogeneity of Ca2+ uptake mediated by the MCU
exists26, and it is still generally assumed that the activity of the Ca2+ conductance associated with the MCU is the same among different tissues27,28. Here we applied the patch-clamp
technique to mitoplasts isolated from a variety of excitable, as well as non-excitable mouse tissues. This method allows for the comparison of MCU activity in different tissues under tightly
controlled conditions of ΔΨm, Ca2+ and H+ gradients across the IMM18. Unexpectedly, of all mouse tissues tested (heart, skeletal muscle, liver, kidney and brown fat), heart mitochondria
manifested the lowest MCU activity. The MCU current density in heart mitochondria was 30 times smaller than that in skeletal muscle. The relevance of this evidence was further corroborated
by our observation that mitochondria from the highly metabolically active _Drosophila_ flight muscle manifests almost no MCU activity, at variance with other populations of _Drosophila_
mitochondria, which indeed possess large current densities. Our results are the first direct evidence that the amount of Ca2+ uptake mediated by the MCU varies between tissues. The low
mitochondrial Ca2+ conductance observed in tissues with a high mitochondrial volume density and that are continuously subjected to high cytosolic Ca2+ elevations, such as mouse heart and
_Drosophila_ flight muscle, is likely to be essential in having normal intracellular Ca2+ signaling, as well as protecting these active tissues from toxic mitochondrial Ca2+ overload.
RESULTS MITOCHONDRIAL CA2+ CURRENT VARIES AMONG MOUSE TISSUES The unique conductance that mediates Ca2+ uptake into mitochondria was shown to be a very selective Ca2+ channel with high
current density in monkey-derived COS7 cells18. In that study, the patch-clamp technique applied to mitoplasts (obtained by hypotonic shock-disruption of the outer mitochondrial membrane
(OMM) of isolated COS7 cell mitochondria) was used to record Ca2+ currents carried by the MCU18. Yet, the success rate in formation of the ‘whole-mitoplast’ mode of the patch-clamp technique
to record currents across the whole native IMM was low. French press employed to mechanically subfractionate mitochondria was advantageous over others methods, as mitoplasts with both
structural and functional integrity were obtained29. Here we patch-clamped mitoplasts derived from French-pressed mitochondria of mouse heart, skeletal muscle, liver, kidney and brown
adipose tissue. The French press protocol used to obtain vescicles of the IMM proved instrumental in increasing the yield of reliable and stable whole-mitoplast recordings from all tissues
studied. Figure 1 (see picture in the inset) shows a differential interference contrast image of a mouse heart mitoplast obtained with the French press method. In 150 mM KCl solution,
mitoplasts generated with the French press method normally have a figure 8-shaped form, as the IMM protrudes through one of small ruptures in the OMM to produce a bilobed vesicle. The lobe
of the mitoplast covered with remnants of the OMM (inset, red arrow) appeared optically denser (as it contained two membranes) than the lobe consisting of the IMM only (inset, white arrow).
After strorage on ice for several hours, mitoplasts assumed a round shape with the remnants of the OMM attached from one side (the so-called ‘cap’ region). We found that the newly prepared
figure 8-shaped mitoplasts were the best choice for the formation of the whole-mitoplast mode, while the success rate in the formation of this mode with older round-shaped mitoplasts was
lower. In the whole-mitoplast configuration, the addition of 100 μM Ca2+ to the bath (the cytosolic face of the IMM) induced an inwardly rectifying Ca2+ current, presumably carried by the
MCU (_I_MCU). _I_MCU current density greatly varied among different tissues examined (Fig. 1). _I_MCU current densities measured upon stepping from 0 mV to −160 mV were as follows: skeletal
muscle, 58±4 pA/pF, _n_=10; brown adipose tissue, 56±7 pA/pF, _n_=6; kidney, 32±3 pA/pF, _n_=10; liver, 25±2 pA/pF, _n_=10; and heart, 2.1±0.6 pA/pF, _n_=8 (Fig. 1, histogram in the lower
right panel, mean±s.e.). Surprisingly, _I_MCU in heart mitoplasts was the lowest among all tissues and as much as 30 times smaller than that in closely related skeletal muscle. In contrast,
another hallmark conductance of the IMM, the inner membrane anion channel30,31,32, was present in all tissues examined (not shown) and showed no difference in activity between heart and
skeletal muscle (Fig. 2). As skeletal muscle and heart are related tissues and showed the higest and lowest _I_MCU current densities, respectively, we compared the biophysical properies of
_I_MCU in these tissues to confirm that _I_MCU has the same properties in heart and skeletal muscle and is thus mediated by the same channel. _I_MCU SHARES THE SAME PROPERTIES IN HEART AND
SKELETAL MUSCLE In both heart and skeletal muscle, _I_MCU elicited by voltage steps was primarily time-independent (Fig. 3a) and apparently lacked Ca2+-dependent inactivation, similar to
what was previously reported for _I_MCU in COS7 cell mitochondria. As expected, in both tissues, _I_MCU was completely blocked by 50 nM RuR, a potent blocker of the MCU17,18 (Fig. 3b). As
expected for the Ca2+ current, _I_MCU gradually increased as Ca2+ concentrations in the bath were gradually elevated from 50 μM to 1 mM in both heart and skeletal muscle (Fig. 4a). Mg2+ did
not induce any _I_MCU (Fig. 4b), and the current traces in the presence of Mg2+ in the bath solution were identical to the baseline (obtained in 200 nM RuR, data not shown). The addition of
Ba2+ to the bath induced _I_MCU, but the Ba2+ current was much smaller than that induced by Ca2+ (Fig. 4b). The permeability sequence for _I_MCU, Ca2+»Ba2+>Mg2+, (Fig. 4c) was the same as
reported for the MCU studied by indirect methods17,33,34 and by patch-clamping mitoplasts from COS7 cells18. Notably, the _I_MCU permeability sequence is unique, as most Ca2+ channels
conduct Ba2+ better than Ca2+ (ref. 35). Ca2+ channels are selective for Ca2+ due to the presence of high-affinity binding sites for Ca2+ in the pore region35. Yet, under divalent-free (DVF)
conditions, monovalent ions, which have significantly lower affinity for these binding sites, can still bind within the pore and permeate the Ca2+ channels35. The monovalent current is
inhibited when Ca2+ is re-added to the bath solution, as Ca2+ occupies the binding site in the pore and thus prevents monovalent ions from permeation. For plasma membrane Ca2+ channels, the
inhibition of the monovalent current by Ca2+ occurs in the low micromolar range, whereas for the MCU of COS7 cells, this inhibition occurs in the low nanomolar range18. This finding
identifies the MCU as the Ca2+ channel with the highest known Ca2+ selectivity. We, therefore, asked whether the MCU in mouse heart and skeletal muscle has the same high selectivity for Ca2+
over monovalent ions as the MCU in COS7 cells. Under DVF conditions when bath and pipette solutions contained 110 mM Na+, we observed a quasi-linear current in both skeletal muscle and
heart (Fig. 5a–c, left panels for skeletal muscle and right panels for heart throughout). This Na+ current was almost completely blocked by as low as 11 nM bath-free Ca2+, and 160 nM Ca2+
totally abolished it (Fig. 5a). The Ca2+-sensitive Na+ current was evidently mediated by the MCU that lost its selectivity under DVF conditions18, which was further confirmed by the fact
that the Na+ current was completely inhibited by 200 nM RuR (Fig. 5b). Similar to COS7 cell mitoplasts18, substitution of Na+ for K+ in the bath solution resulted in significantly lower
monovalent current through the MCU, showing that the MCU was relatively impermeant to K+ ions, in both skeletal muscle and heart (Fig. 5c). The amplitude of the Na+ current through the MCU
was dramatically lower in heart (23±1 pA/pF, _n_=5) compared with skeletal muscle (713±23 pA/pF, _n_=5), which correlates well with the difference in amplitude of the Ca2+ currents between
these two tissues (Fig. 5d). We conclude that _I_MCU shares the same biophysical properties in heart and skeletal muscle, and these properties coincide with those of _I_MCU in COS7 cells18.
Thus _I_MCU in heart and skeletal muscle is likely mediated by the same molecule, but heart mitochondria are endowed with dramatically lower MCU activity. _I_MCU IS SIGNIFICANTLY LARGER IN
NEONATAL VERSUS ADULT HEART An important unresolved problem in cardiac Ca2+ signalling is whether beat-to-beat elevations of mitochondrial [Ca2+] occur in intact cardiomyocytes. Although
this issue was addressed by several laboratories, a general consensus has not been reached. In a recent review, it has been noted that beat-to-beat oscillations might be developmentally
regulated and occur in neonatal but not adult cardiomyocytes7. Although it has never been previously suggested, one of the specific reasons for this may be a higher Ca2+ conductance of the
IMM in neonatal cardiomyocytes as compared with adult cells. To test this hypothesis, we compared _I_MCU current density in cardiac mitoplasts isolated from newborn (2-day-old) and adult
(>3 weeks) mice. Whole-mitoplast Ca2+ currents from 2-day-old heart were recorded using the same solutions and voltage protocols used for adult heart. Interestingly, _I_MCU current
density in neonatal mitoplasts measured with 100 μM Ca2+ in the bath was five times larger (10.2±0.3 pA/pF) than in adult mitoplasts (2.1±0.5 pA/pF, see Fig. 6). These results demonstrate
that the IMM of the neonatal heart has significantly larger Ca2+ conductance as compared with adult heart, which may explain the age-related differences in beat-to-beat mitochondrial Ca2+
accumulation in cardiomyocytes. TISSUE HETEROGENEITY OF _I_MCU IN _DROSOPHILA_ Similar to our findings in mouse tissues, we found a dramatic difference in the density of the mitochondrial
Ca2+ current among _Drosophila_ tissues. We first studied Ca2+ currents in mitoplasts isolated from _Drosophila_ flight muscle, which is of particular interest because this organ contracts
at high frequencies. We obtained mitoplasts from thoraces of a _Drosophila_ line in which the targeted mitochondrial expression of green fluorescent protein (GFP) in muscles was driven by
crossing a upstream activating sequence-MitoGFP line with a muscle specific Mef2-Gal4 line (see Methods for details). Flight muscle mitochondria from this line express GFP in the matrix;
hence, mitoplasts originating from it fluoresce. _Drosophila_ thoraces were cleaned of legs to reduce contamination from leg muscle mitochondria. Figure 7a shows a typical flight muscle
GFP-fluorescent mitoplast used for patch-clamp recordings. Similar to mouse mitoplasts, _Drosophila_ mitoplasts were obtained using the French press procedure and normally showed the same
figure 8-shaped morphology (compare Figs 7a and 1 inset). The lobe that contained only the IMM was distinguishable from the optically denser lobe that was covered with the OMM and contained
two membranes (Fig. 7a, white and red arrows, respectively). _Drosophila_ flight muscle whole-mitoplast Ca2+ currents were recorded using the same solutions and voltage protocols used for
mouse mitoplasts. Interestingly, the Ca2+ current induced by the gradual elevation of Ca2+ in the bath from 100 μM to 5 mM was barely detectable (Fig. 7b, left panel). Similar to the MCU
current observed in mammalian mitoplasts, this small Ca2+ current was RuR sensitive (Fig. 7b, left panel). In contrast, when we specifically selected non-GFP mitoplasts that originated from
tissues other than flight muscle of the Mef2 >MitoGFP flies, we were able to record robust whole-mitoplast RuR-sensitive Ca2+ currents. Figure 7b shows a representative Ca2+ current
recorded from one such mitoplast. Interestingly, the density of the Ca2+ current recorded from this particular mitoplast at 100 μM Ca2+ (175 pA/pF) was significantly higher than that in any
other mouse tissue examined (Fig. 1). Overall, we recorded 31 _Drosophila_ mitoplasts. Those mitoplasts originating from the flight muscle (_n_=21) consistently showed a very-low Ca2+
current both in 100 μM Ca2+ (0.4±0.2 pA/pF, _n_=8) and in 1 mM Ca2+ (3.9±0.1 pA/pF, _n_=13). Among the 10 mitoplasts derived from tissues other than flight muscle, six showed a Ca2+ current
significantly higher than that observed in flight muscle and four showed a very small Ca2+ current. The six _Drosophila_ mitoplasts with high Ca2+ currents had very different values for
current density (from 15 to 175 pA/pF in 100 μM Ca2+ and from 11 to 800 pA/pF in 1 mM Ca2+). The densities of the mitochondrial Ca2+ currents from _Drosophila_ flight muscle and other
tissues are compared in Fig. 7c. In spite of the fact that the amplitude of the Ca2+ current was extremely low in flight muscle mitoplasts, these mitoplasts had very large Cl− current (Fig.
8) with the mean current density of 161±6 pA/pF at +80 mV, _n_=4. This is the first observation of the existence of a mitochondrial Cl− conductance in _Drosophila_. Therefore, as seen for
mouse tissues, _Drosophila_ tissues display significant differences in the mitochondrial Ca2+ current density. RuR-sensitive Ca2+ uptake into mitochondria isolated from the
_Drosophila_-derived S2 cell line has recently been demonsrated36. This uptake and the RuR-sensitive Ca2+ currents recorded from _Drosophila_ mitoplasts are both likely to be mediated by a
_Drosophila_ homologue of the mammalian MCU. DISCUSSION The phenomenon of MCU-mediated Ca2+ uptake is one of the most interesting aspects of mitochondrial physiology. From its original
discovery in the early 1960s (refs 37,38), this process was studied primarily with biochemical (ion flux studies) and optical (fluorescent proteins and indicators) methods, in either
suspensions of isolated mitochondria or intact cells3,17,23,25. Although these methods identified the phenomenon of mitochondrial Ca2+ uptake and provided its initial characterization, they
did not allow for the reliable control of experimental conditions, and there have been significant variations in the rate of mitochondrial uptake measured in the same tissues by different
labs17,23,25. Reliable comparisons of mitochondrial Ca2+ uptake between different tissues have been difficult, and rare reports on the tissue-specific differences in MCU activity26 have also
been largely overlooked. Therefore, it was eventually concluded that mammalian mitochondria have a high rate of Ca2+ uptake, and the MCU was described as a ‘high-capacity’ Ca2+ transport
mechanism17,23. Indeed, in many tissues, mitochondrial Ca2+ uptake potently affects cytosolic Ca2+ transients and causes a significant elevation of Ca2+ in the mitochondrial matrix10,24.
Thus, the present consensus in the field is that all mammalian mitochondria are endowed with approximately the same MCU activity and the same high ability to accumulate Ca2+. The
demonstration that whole-IMM and unitary MCU currents can be recorded with the patch-clamp technique18 overcame the technical limitations of the biochemical and optical methods. For the
first time, this allowed direct studies of MCU activity under highly controlled conditions, such as exact potential across the IMM and exact concentrations for all ions on both sides of the
IMM. Importantly, the patch-clamp technique allows for the dissection of single mechanisms involved in mitochondrial Ca2+ transport and allows the characterization of MCU activity at the
single organelle level. Here we applied the patch-clamp technique to compare the properties of Ca2+ currents carried by the MCU in different mouse tissues. This study unexpectedly showed
that the density of the MCU current in mouse heart is significantly lower than that in other mouse tissues. Interestingly, the MCU current density in heart was 30 times lower than that in
closely related skeletal muscle. This dramatic difference is no likely the result of differences in the molecules responsible for mitochondrial Ca2+ uptake in heart and skeletal muscle, as
key biophysical properties of the mitochondrial Ca2+ current in heart and skeletal muscle were the same and correlated well with the biophysical properties of the MCU in COS7 cells18. The
very low density of the MCU current in heart was an unexpected finding, as accumulation of Ca2+ into mitochondria in intact cardiac muscle has been previously demonstrated6,7,8,39, and
mitochondrial Ca2+ uptake in metabolically active tissues such as heart is perceived as important for the stimulation of mitochondrial ATP production and matching its rate with cytosolic
Ca2+ activity7,8,10. The paradoxically low MCU activity in heart, however, can be explained if we consider that mitochondria occupy up to 37% of the total cell volume in heart40. If these
many heart mitochondria possessed a high ability to accumulate Ca2+, they could abolish the cytosolic Ca2+ signalling necessary for heart contractile activity. Furthermore, because heart is
characterized by frequent elevations of cytosolic Ca2+, robust mitochondrial Ca2+ uptake would lead to extensive futile cycling of Ca2+ across the IMM, resulting in low metabolic efficiency.
Finally, the frequent elevation of cytosolic Ca2+ could lead to mitochondrial Ca2+ overload and dysfunction. Therefore, in heart, with its abundant mitochondria and frequent elevations in
cytosolic Ca2+, it may be essential to limit MCU activity to preserve both a normal Ca2+-dependent heartbeat and mitochondrial integrity. In contrast, because skeletal muscle mitochondria
occupy only about 5% of the cell volume41,42 and are not exposed to frequent Ca2+ elevations, limiting MCU activity in this tissue might not be necessary. It must be noted that although
singular heart mitochondria have a limited ability to uptake Ca2+, their abundance can result in combined Ca2+ accumulation comparable to other tissues. Interestingly, the density of the MCU
current in cardiac mitoplasts isolated from newborn mice was about five times larger than that of adult mice. This demonstrates that the MCU activity can vary significantly not only between
tissues but also during development. Important morphological changes occur during the first week after birth in cardiac myocytes, and the SR and mitochondrial networks are extensively
remodeled7. In particular, mitochondrial volume density increases from about 20% in newborn to about 40% adults43,44. The age-related differences in the Ca2+ conductance of the cardiac IMM
reported here, together with the morphological changes in SR and mitochondrial network, may explain why beat-to-beat oscillations of mitochondrial Ca2+ are usually observed in newborn and
less often in adult cardiomyocytes7,8. _Drosophila_ flight muscle is characterized by even higher Ca2+-dependent contractile activity than mouse heart, and mitochondria in this tissue occupy
about 40% of the cell volume45,46. Therefore, it is not surprising that the MCU current density in _Drosophila_ flight muscle is about four times lower than that in mouse heart. However,
some mitoplasts originating from _Drosophila_ tissues other than flight muscle have very high MCU current density, even exceeding the maximal MCU current density found in mice. Thus, in
_Drosophila_, as seen in mice, MCU activity varies dramatically between tissues. Whether variations in MCU activity are due to tissue-specific regulation of the MCU (for example, via
auxiliary subunits) or to different tissue-specific expression of the MCU channel requires further investigation. However, the tight control of MCU activity in highly active cells with
intensive cytosolic Ca2+ signalling is likely crucial for the normal physiology of these cells and for the integrity of their mitochondria. Misregulation of MCU activity in such tissues may
lead to derangement of intracellular Ca2+ signalling and mitochondrial dysfunction, followed by functional incompetence and degeneration. METHODS ISOLATION OF MOUSE MITOPLASTS FOR
PATCH-CLAMP RECORDINGS Mitoplasts were prepared from heart, skeletal muscle, liver, kidney and brown fat of adult (3–6-week-old) mice. Mitoplasts were isolated from wild-type C56BL/6 mice,
except for brown fat mitoplasts obtained from mice deficient for uncoupling protein 1 (UCP1 −/−, C56BL/6 background, courtesy of Dr. Leslie Kozak, Pennington Biomedical Research Center,
Louisiana State University, Baton Rouge, Louisiana, USA). Mice were killed by CO2 asphyxiation followed by cervical dislocation. The selected mouse tissue was isolated, rinsed and
homogenized in ice-cold medium containing 250 mM sucrose, 10 mM HEPES, and 1 mM EGTA (pH adjusted to 7.25 with KOH), using a glass grinder with six slow strokes of a Teflon pestle rotating
at 280 (soft tissues) or 600 (fibrous tissues) rotations per minute. The homogenate was centrifuged at 700 _g_ for 5–10 min to pellet nuclei and unbroken cells. For some tissues, the first
nuclear pellet was resuspended in the same solution and homogenized again to increase the yield of mitochondria. Mitochondria were collected by centrifugation of the supernatant at 8,500 _g_
for 10 min. Mitoplasts were produced from mitochondria using a French press. Briefly, mitochondria were suspended in a solution containing 140 mM sucrose, 440 mM D-mannitol, 5 mM HEPES, and
1 mM EGTA (pH adjusted to 7.2 with KOH), and then subjected to a French press at 2,000 psi to rupture the outer membrane. Mitoplasts were pelleted at 10,500 _g_ for 15 min and resuspended
for storage in 500 μl of solution containing 750 mM KCl, 100 mM HEPES and 1 mM EGTA (pH adjusted to 7.2 with KOH). Mitochondria and mitoplasts were prepared at 0–4 °C and stored on ice for
up to 5 h. The French press method allows for a more gentle mechanical isolation of mitoplasts, and the pressure applied can be controlled to preserve the cristae of mitoplasts29.
Immediately before the electrophysiological experiments, 15–50 μl of the mitoplast suspension was added to 500 μl of a solution containing 150 mM KCl, 10 mM HEPES and 1 mM EGTA (pH adjusted
to 7.2 with KOH), and plated on 5-mm coverslips. For whole-mitoplast experiments, coverslips were pretreated with 0.1% gelatin to reduce adhesion. Only mitoplasts that had a figure 8-shaped
appearance were selected for experiments, as this indicated the integrity of the matrix and membranes (Fig. 1). ISOLATION OF _DROSOPHILA_ MITOPLASTS The upstream activating
sequence-MitoGFP47,48 and Mef2-Gal4 (refs 47,49) flies were used as previously described. Flies were raised at 25 °C, and adult flies >5 days of age after eclosion were used. _Drosophila_
mitoplasts were obtained from either the combined thoraces (devoid of legs and wings) of 70 flies or 30–50 whole-bodies of the Mef2 >MitoGFP flies. After grinding thoraces/whole flies
with a Politron homogenizer for 3 min (rheostat set at 4), mitoplasts were obtained with the same protocol used for mouse mitoplasts (see above). Note that French-pressed _Drosophila_
mitoplasts maintained the same figure 8-shaped morphology as seen in mouse mitoplasts (compare Figs 7a and 1 inset, white arrows). PATCH-CLAMP RECORDINGS Mouse or _Drosophila_ mitoplasts
(2–6 μm in size) were used for patch-clamp experiments and typically had membrane capacitances of 0.3–1.3 pF. Currents were recorded using an Axopatch 200B amplifier (Molecular Devices). The
voltage protocols and recording conditions are as indicated in the figures. Mitoplasts were stimulated every 5 s. To minimize errors in the voltage clamp associated with the liquid junction
potential, a 3-M KCl-agar salt bridge was used as the bath reference electrode. Gigaohm seals with mitoplasts were formed in the bath solution containing 150 mM KCl, 10 mM HEPES and 1 mM
EGTA, pH 7.2 (adjusted with KOH). Capacitative transients were completely compensated right after the seal was formed. Voltage steps of 350–500 mV from 15–50 ms were applied to rupture the
IMM and obtain the whole-mitoplast configuration, as monitored by the appearance of capacitance transients and an increase in baseline noise. The access resistance and membrane capacitance
of mitoplasts were determined using the Membrane Test tool of pClamp 10 (Molecular Devices). Typically, pipettes had resistances of 30–50 MΩ, and the access resistance was 40–80 MΩ. The
averaged membrane capacitance of mitoplasts used in our experiments varied between tissues and was (0.65±0.04 pF, _n_=40) for skeletal muscle, (0.68±0.04 pF, _n_=13) for brown fat,
(0.54±0.02 pF, _n_=18) for kidney, (0.36±0.02 pF, _n_=19) for liver, (0.67±0.02 pF, _n_=55) for heart and (1.31±0.06 pF, _n_=54) for _Drosophila_ flight muscle. The calculated voltage clamp
errors associated with the access resistance never exceeded 10 mV. Current amplitudes for histograms were measured within 5 ms after stepping the membrane from 0 to −160 mV. To measure Ca2+
currents through the MCU, Figs 1, 2, 3, 5 and 6, pipettes were filled with 150 mM tetramethylammonium hydroxide, 1.5 mM EGTA, 1 mM MgCl2 (2 mM MgCl2 for _Drosophila_), and 150 mM HEPES, pH
7.0 with D-gluconic acid, tonicity 450 mmol per kg (~330 mmol per kg for _Drosophila_) with sucrose. In some experiments for _Drosophila_ recordings, the tetramethylammonium hydroxide
pipette solution was replaced with 110 mM Na-gluconate, 40 mM HEPES, 1.5 mM EGTA and 2 mM NaCl (pH adjusted to 7.0 with NaOH, tonicity adjusted to ~330 mmol per kg with sucrose).
Whole-mitoplast control currents in Figs 1, 2, 3a, 5, were recorded in a bath solution (HEPES/Tris solution) containing 150 mM HEPES and 1 mM EGTA (pH adjusted to 7.0 with Tris-base,
tonicity adjusted to ~300 mmol per kg with sucrose). To record whole-mitoplast _I_MCU Ca2+ currents (Figs 1, 2, 3, 5 and 6), a 1-M CaCl2 stock solution was diluted to the final desired
concentration in the HEPES/Tris solution without EGTA. To block _I_MCU Ca2+ currents, RuR was added to the 100-μM Ca2+ bath solution at a final concentration of 50 nM (Fig. 7a) or to the 1–5
mM Ca2+ bath solution at a final concentration of 200 nM (left and right panels of Fig. 7b). Bath solutions containing 100 μM–1 mM Ba2+ or Mg2+ were obtained by the addition of a 1-M stock
solution of the corresponding chloride salt into the HEPES-Tris solution without EGTA. To study the monovalent current carried by MCU in DVF conditions (Fig. 5), the following pipette
solution was used: 110 mM Na-gluconate, 40 mM HEPES, 1 mM EGTA, 5 mM EDTA and 2 mM NaCl (pH adjusted to 7.0 with NaOH, tonicity adjusted to ~450 mmol per kg with sucrose). Bath solution used
for these experiments contained: 110 mM Na-gluconate, 40 mM HEPES, 1 mM EGTA and 5 mM EDTA (pH 7.0 with NaOH, tonicity adjusted to 300 mmol per kg with sucrose). CaCl2 was added to the
Na-gluconate bath solution in the amount calculated by the MaxChelator winMaxC v2.05 programme (C. Patton, Stanford University) to obtain various free concentrations of Ca2+ in the nanomolar
range (Fig. 4a). For experiments in which we studied the permeability of the MCU to K+ in the DVF conditions (Fig. 4c), the following bath solution was employed: 110 mM K-gluconate, 40 mM
HEPES, 1 mM EGTA and 5 mM EDTA (pH 7.0 with KOH, tonicity adjusted to 300 mmol per kg with sucrose). All electrophysiological experiments were performed under continuous perfusion of the
bath solution. Data acquisition and analyses were performed using PClamp 10 (Molecular Devices) and Origin 7.5 (OriginLab). All electrophysiological data presented were acquired at 10 kHz
and filtered at 1 kHz. Statistical data are presented as the mean±s.e. All general chemicals as were purchased from Sigma (USA). ADDITIONAL INFORMATION HOW TO CITE THIS ARTICLE: Fieni, F.
_et al_. Activity of the Mitochondrial Calcium Uniporter Varies Greatly between Tissues. _Nat. Commun._ 3:1317 doi: 10.1038/ncomms2325 (2012). REFERENCES * Duchen M. R. Contributions of
mitochondria to animal physiology: from homeostatic sensor to calcium signalling and cell death. _J. Physiol._ 516 (Pt 1): 1–17 (1999). Article CAS Google Scholar * Carafoli E. Calcium
signaling: a tale for all seasons. _Proc. Natl Acad. Sci. USA_ 99, 1115–1122 (2002). Article ADS CAS Google Scholar * Carafoli E. The interplay of mitochondria with calcium: An
historical appraisal. _Cell Calcium_ 52, 1–8 (2012). Article CAS Google Scholar * McCormack J. G., Halestrap A. P., Denton R. M. Role of calcium ions in regulation of mammalian
intramitochondrial metabolism. _Physiol. Rev._ 70, 391–425 (1990). Article CAS Google Scholar * Hajnoczky G., Robb-Gaspers L. D., Seitz M. B., Thomas A. P. Decoding of cytosolic calcium
oscillations in the mitochondria. _Cell_ 82, 415–424 (1995). Article CAS Google Scholar * Robert V. et al. Beat-to-beat oscillations of mitochondrial [Ca2+] in cardiac cells. _EMBO J._
20, 4998–5007 (2001). Article CAS Google Scholar * Griffiths E. J., Balaska D., Cheng W. H. The ups and downs of mitochondrial calcium signalling in the heart. _Biochim. Biophys. Acta._
1797, 856–864 (2010). Article CAS Google Scholar * Dedkova E. N., Blatter L. A. Mitochondrial Ca2+ and the heart. _Cell Calcium_ 44, 77–91 (2008). Article CAS Google Scholar * Nicholls
D. G. Mitochondrial calcium function and dysfunction in the central nervous system. _Biochim. Biophys. Acta._ 1787, 1416–1424 2009. Article CAS Google Scholar * Rizzuto R., Bernardi P.,
Pozzan T. Mitochondria as all-round players of the calcium game. _J. Physiol._ 529(Pt 1): 37–47 (2000). Article CAS Google Scholar * Carafoli E., Patriarca P., Rossi C. S. A comparative
study of the role of mitochondria and the sarcoplasmic reticulum in the uptake and release of Ca2+ by the rat diaphragm. _J. Cell. Physiol._ 74, 17–30 (1969). Article CAS Google Scholar *
Rudolf R., Mongillo M., Magalhaes P. J., Pozzan T. _In vivo_ monitoring of Ca2+ uptake into mitochondria of mouse skeletal muscle during contraction. _J. Cell. Biol._ 166, 527–536 (2004).
Article CAS Google Scholar * Quintana A., Hoth M. Mitochondrial dynamics and their impact on T cell function. _Cell Calcium_ 52, 57–63 (2012). Article CAS Google Scholar * Halestrap A.
P. Calcium, mitochondria and reperfusion injury: a pore way to die. _Biochem. Soc. Trans._ 34, 232–237 (2006). Article CAS Google Scholar * Pacher P., Hajnoczky G. Propagation of the
apoptotic signal by mitochondrial waves. _EMBO J._ 20, 4107–4121 (2001). Article CAS Google Scholar * Carafoli E. Intracellular calcium homeostasis. _Annu. Rev. Biochem._ 56, 395–433
(1987). Article CAS Google Scholar * Gunter T. E., Pfeiffer D. R. Mechanisms by which mitochondria transport calcium. _Am. J. Physiol._ 258, C755–C786 (1990). Article CAS Google Scholar
* Kirichok Y., Krapivinsky G., Clapham D. E. The mitochondrial calcium uniporter is a highly selective ion channel. _Nature_ 427, 360–364 (2004). Article ADS CAS Google Scholar * De
Stefani D., Raffaello A., Teardo E., Szabo I., Rizzuto R. A forty-kilodalton protein of the inner membrane is the mitochondrial calcium uniporter. _Nature_ 476, 336–340 (2011). Article ADS
CAS Google Scholar * Baughman J. M. et al. Integrative genomics identifies MCU as an essential component of the mitochondrial calcium uniporter. _Nature_ 476, 341–345 (2011). Article
ADS CAS Google Scholar * Perocchi F. et al. MICU1 encodes a mitochondrial EF hand protein required for Ca2+ uptake. _Nature_ 467, 291–296 (2010). Article ADS CAS Google Scholar *
Mallilankaraman K. et al. MICU1 is an essential gatekeeper for MCU-mediated mitochondrial Ca(2+) uptake that regulates cell survival. _Cell_ 151, 630–644 (2012). Article CAS Google Scholar
* Bernardi P. Mitochondrial transport of cations: channels, exchangers, and permeability transition. _Physiol. Rev._ 79, 1127–1155 (1999). Article CAS Google Scholar * Rizzuto R.,
Simpson A. W., Brini M., Pozzan T. Rapid changes of mitochondrial Ca2+ revealed by specifically targeted recombinant aequorin. _Nature_ 358, 325–327 (1992). Article ADS CAS Google Scholar
* Pozzan T., Rudolf R. Measurements of mitochondrial calcium _in vivo_. _Biochim. Biophys. Acta._ 1787, 1317–1323 (2009). Article CAS Google Scholar * Nicholls D. G. Calcium transport
and porton electrochemical potential gradient in mitochondria from guinea-pig cerebral cortex and rat heart. _Biochem. J._ 170, 511–522 (1978). Article CAS Google Scholar * Chalmers S.,
Nicholls D. G. The relationship between free and total calcium concentrations in the matrix of liver and brain mitochondria. _J. Biol. Chem._ 278, 19062–19070 (2003). Article CAS Google
Scholar * Brookes P. S. et al. UCPs–unlikely calcium porters. _Nat. Cell. Biol._ 10, 1235–1237 (2008). Article CAS Google Scholar * Decker G. L., Greenawalt J. W. Ultrastructural and
biochemical studies of mitoplasts and outer membranes derived from French-pressed mitochondria. Advances in mitochondrial subfractionation. _J. Ultrastruct. Res._ 59, 44–56 (1977). Article
CAS Google Scholar * Sorgato M. C., Keller B. U., Stuhmer W. Patch-clamping of the inner mitochondrial membrane reveals a voltage-dependent ion channel. _Nature_ 330, 498–500 (1987).
Article ADS CAS Google Scholar * Borecky J., Jezek P., Siemen D. 108-pS channel in brown fat mitochondria might Be identical to the inner membrane anion channel. _J. Biol. Chem._ 272,
19282–19289 (1997). CAS PubMed Google Scholar * Garlid K. D., Beavis A. D. Evidence for the existence of an inner membrane anion channel in mitochondria. _Biochim. Biophys. Acta._ 853,
187–204 (1986). Article CAS Google Scholar * Drahota Z., Gazzotti P., Carafoli E., Rossi C. S. A comparison of the effects of different divalent cations on a number of mitochondrial
reactions linked to ion translocation. _Arch. Biochem. Biophys._ 130, 267–273 (1969). Article CAS Google Scholar * Vainio H., Mela L., Chance B. Energy dependent bivalent cation
translocation in rat liver mitochondria. _Eur. J. Biochem._ 12, 387–391 (1970). Article CAS Google Scholar * Hille B. _Ionic Channels Of Excitable Membranes_ 2nd edn Sinauer Associates
(1992). * von Stockum S. et al. Properties of Ca(2+) transport in mitochondria of Drosophila melanogaster. _J. Biol. Chem._ 286, 41163–41170 (2011). Article CAS Google Scholar * Deluca H.
F., Engstrom G. W. Calcium uptake by rat kidney mitochondria. _Proc. Natl Acad. Sci. USA_ 47, 1744–1750 (1961). Article ADS CAS Google Scholar * Vasington F. D., Murphy J. V. Ca ion
uptake by rat kidney mitochondria and its dependence on respiration and phosphorylation. _J. Biol. Chem._ 237, 2670–2677 (1962). CAS PubMed Google Scholar * Maack C. et al. Elevated
cytosolic Na+ decreases mitochondrial Ca2+ uptake during excitation-contraction coupling and impairs energetic adaptation in cardiac myocytes. _Circ. Res._ 99, 172–182 (2006). Article CAS
Google Scholar * Barth E., Stammler G., Speiser B., Schaper J. Ultrastructural quantitation of mitochondria and myofilaments in cardiac muscle from 10 different animal species including
man. _J. Mol. Cell. Cardiol._ 24, 669–681 (1992). Article CAS Google Scholar * Drexler H. et al. Alterations of skeletal muscle in chronic heart failure. _Circulation_ 85, 1751–1759
(1992). Article CAS Google Scholar * Hoppeler H., Fluck M. Plasticity of skeletal muscle mitochondria: structure and function. _Med. Sci. Sports Exerc._ 35, 95–104 (2003). Article CAS
Google Scholar * Olivetti G., Anversa P., Melissari M., Loud A. V. Morphometric study of early postnatal development of the thoracic aorta in the rat. _Circ. Res._ 47, 417–424 (1980).
Article CAS Google Scholar * Legato M. J. Cellular mechanisms of normal growth in the mammalian heart. II. A quantitative and qualitative comparison between the right and left ventricular
myocytes in the dog from birth to five months of age. _Circ. Res._ 44, 263–279 (1979). Article CAS Google Scholar * Magwere T. et al. The effect of dietary restriction on mitochondrial
protein density and flight muscle mitochondrial morphology in Drosophila. _J. Gerontol. A. Biol. Sci. Med. Sci._ 61, 36–47 (2006). Article Google Scholar * Suarez R. K., Lighton J. R.,
Brown G. S., Mathieu-Costello O. Mitochondrial respiration in hummingbird flight muscles. _Proc. Natl Acad. Sci. USA_ 88, 4870–4873 (1991). Article ADS CAS Google Scholar * Park J. et
al. Mitochondrial dysfunction in Drosophila PINK1 mutants is complemented by parkin. _Nature_ 441, 1157–1161 (2006). Article ADS CAS Google Scholar * Verstreken P. et al. Synaptic
mitochondria are critical for mobilization of reserve pool vesicles at Drosophila neuromuscular junctions. _Neuron_ 47, 365–378 (2005). Article CAS Google Scholar * Ranganayakulu G. et
al. A series of mutations in the D-MEF2 transcription factor reveal multiple functions in larval and adult myogenesis in Drosophila. _Dev. Biol._ 171, 169–181 (1995). Article CAS Google
Scholar Download references ACKNOWLEDGEMENTS This work was funded by the NIH Director’s New Innovator Award DP2OD004656-01. AUTHOR INFORMATION Author notes * Sung Bae Lee Present address:
Present address: Department of Brain Science, Daegu Gyeongbuk Institute of Science and Technology, Daegu 711-873, Korea, AUTHORS AND AFFILIATIONS * Department of Physiology, University of
California, San Francisco, UCSF Mail Code 2140, Genentech Hall Room N272F, 600 16th Street, San Francisco, 94158, California, USA Francesca Fieni, Sung Bae Lee, Yuh Nung Jan & Yuriy
Kirichok * Department of Physiology, Howard Hughes Medical Institute, University of California, San Francisco, Room 484E, 1550 4th Street, San Francisco, 94158, California, USA Sung Bae Lee
& Yuh Nung Jan Authors * Francesca Fieni View author publications You can also search for this author inPubMed Google Scholar * Sung Bae Lee View author publications You can also search
for this author inPubMed Google Scholar * Yuh Nung Jan View author publications You can also search for this author inPubMed Google Scholar * Yuriy Kirichok View author publications You can
also search for this author inPubMed Google Scholar CONTRIBUTIONS F.F. and Y.K. conceived the project, designed the experiments, and wrote the manuscript. F.F. performed all
electrophysiological experiments. S.B.L. and Y.N.J. generated the _Drosophila_ line expressing mitochondria GFP in the flight muscle. All authors discussed the results and commented on the
manuscript. CORRESPONDING AUTHOR Correspondence to Yuriy Kirichok. ETHICS DECLARATIONS COMPETING INTERESTS The authors declare no competing financial interests. RIGHTS AND PERMISSIONS
Reprints and permissions ABOUT THIS ARTICLE CITE THIS ARTICLE Fieni, F., Bae Lee, S., Jan, Y. _et al._ Activity of the mitochondrial calcium uniporter varies greatly between tissues. _Nat
Commun_ 3, 1317 (2012). https://doi.org/10.1038/ncomms2325 Download citation * Received: 27 June 2012 * Accepted: 20 November 2012 * Published: 27 December 2012 * DOI:
https://doi.org/10.1038/ncomms2325 SHARE THIS ARTICLE Anyone you share the following link with will be able to read this content: Get shareable link Sorry, a shareable link is not currently
available for this article. Copy to clipboard Provided by the Springer Nature SharedIt content-sharing initiative