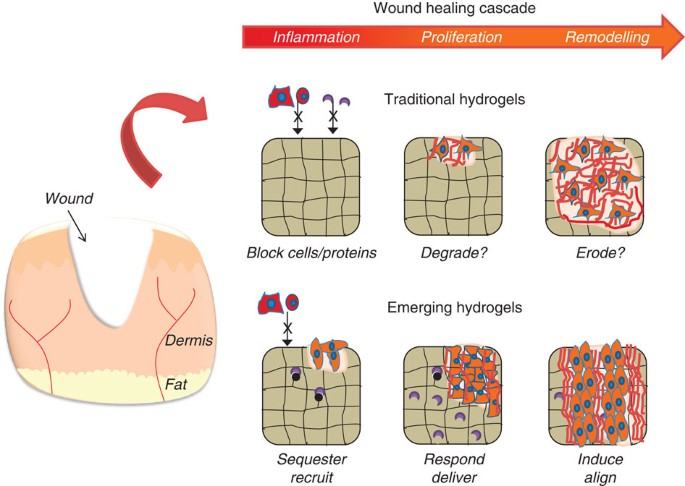
- Select a language for the TTS:
- UK English Female
- UK English Male
- US English Female
- US English Male
- Australian Female
- Australian Male
- Language selected: (auto detect) - EN
Play all audios:
ABSTRACT Hydrogels are water-swollen polymer networks that have found a range of applications from biological scaffolds to contact lenses. Historically, their design has consisted primarily
of static systems and those that exhibit simple degradation. However, advances in polymer synthesis and processing have led to a new generation of dynamic systems that are capable of
responding to artificial triggers and biological signals with spatial precision. These systems will open up new possibilities for the use of hydrogels as model biological structures and in
tissue regeneration. You have full access to this article via your institution. Download PDF SIMILAR CONTENT BEING VIEWED BY OTHERS MODEL-BASED MODULAR HYDROGEL DESIGN Article 26 March 2024
DESIGN PRINCIPLES FOR STRONG AND TOUGH HYDROGELS Article 07 May 2024 RAPID FABRICATION OF PHYSICALLY ROBUST HYDROGELS Article 21 August 2023 INTRODUCTION Hydrogels are water-swollen polymer
networks that have been used for many decades, with applications as varied as contact lenses and super-absorbant materials. As the field of biomedical engineering has developed, hydrogels
have become a prime candidate for application as molecule delivery vehicles and as carriers for cells in tissue engineering, owing to their ability to mimic many aspects of the native
cellular environment (for example, high water content, mechanical properties that match soft tissues). Traditional hydrogels, formed through the covalent and non-covalent crosslinking of
polymer chains, were regarded as relatively inert materials, providing a simple biomimetic three-dimensional (3D) environment, either for tissue production by local resident cells or for
positioning of cells delivered _in vivo_. However, the simplicity of these materials may have in fact hindered their application, restricting cellular interactions with the environment and
preventing uniform extracellular matrix (ECM) production and proper tissue development. In addition, these materials were limited to modelling static environments and lacked the
spatiotemporal dynamic properties relevant for complex tissue processes. Fortunately, during the last decade the concepts of hydrogel design and cellular interaction have evolved, shedding
light on how they may control cell behaviour, particularly for tissue engineering applications. Hydrogels with a range of mechanical properties, and capable of incorporating a wide range of
biologically relevant molecules, from individual functional groups to multidomain proteins, are currently in development1. In addition, hydrogels are being designed with spatial
heterogeneity, to either replicate properties in native tissue structures or to produce constructs with distinct regionally specific cell behavior2. As a result, studies to date have clearly
demonstrated the possibility of creating well-defined microenvironments with control over the 3D presentation of signals to cells3. However, recently there has been a focus on the concept
of hydrogels that exhibit dynamic complexity. These materials should evolve with time and in response to user-defined triggers or cellular behaviour. Indeed, a cadre of recent examples
demonstrates that hydrogels can be used as well-defined static platforms for presenting signals to cells, and furthermore as dynamic evolving environments. It is too soon to tell how closely
these materials mimic the dynamic complexity of the cell/hydrogel interface, or that of multicellular constructs or cell condensates, or to what extent dynamic hydrogels can regulate
emergent biological processes such as tissue development. However, it is clear that increased material complexity is beginning to address such questions. The objective of this review is to
highlight the evolution of hydrogel design towards dynamic behaviour. We first consider stable and patterned hydrogels, then hydrogels that undergo either hydrolytic or proteolytic
degradation, and finally describe hydrogels with trigger-responsive properties. We particularly emphasize recent developments in hydrogel design that offer the ability to precisely control
cell–microenvironment interactions, such as those found in cellular processes. STATIC HYDROGELS THAT MIMIC BIOPHYSICAL CUES We are constantly improving our understanding of biochemical and
physicochemical signals in the local cellular microenvironment, and their role in cellular signalling. This has been difficult for natural materials, where many cues (for example, adhesive
and mechanical) are coupled, yet synthetic hydrogels have provided a well-defined platform for experimentation. A variety of biochemical signals have been explored upon or within hydrogels,
taking advantage of hydrogels as ‘blank slates’ that can be decorated with cell-interactive4, or growth factor-binding5,6,7, ligands. Hydrogels are also useful because their physical
properties are biomimetic, that is, they can be designed with stiffness ranges and topographical features that are analogous to natural extracellular environments8. As an example, 2D
polyacrylamide gels with tunable mechanical properties have provided a platform for investigating mechanotransduction behavior9,10, suggesting that stem cell fate is strongly influenced by
the mechanics of the target tissue11. Additional related studies have emphasized the critical role of hydrogel stiffness in skeletal muscle stem cell self-renewal12, neural stem cell
behavior13 and megakaryocyte poiesis14. 3D mechanical properties are similarly important in controlling adult stem cell fate in alginate hydrogels incorporating adhesive ligands4. In this
case, hydrogel mechanics alter the ability of a cell to cluster tethered ligands and exert tension, leading to different fate decisions (for example, adipogenic fate in softer gels and
osteogenic fate in stiffer gels). Several further examples also demonstrate that 3D hydrogels support the growth of a wide variety of tissues15; however, the synthesized tissue properties
are often inferior to native tissue, for a range of reasons possibly related to the simplicity of these basic designs. These studies illustrate that static hydrogels are a useful,
well-defined platform to efficiently probe how cell behaviour is influenced by the microenvironment, or by defined physicochemical and biological inputs. However, biological systems are
rarely static and homogeneous, and there is a wealth of biology and a cadre of intriguing hypotheses to be addressed with increasing complexity in hydrogel design. INTRODUCING SPATIAL
HETEROGENEITY INTO HYDROGELS Many biological processes are heterogeneous and material design is evolving to emulate them. An example of wound healing from the perspective of traditional
static versus emerging hydrogels is illustrated in Fig. 1. Wound healing is a complex process that involves spatial and temporal heterogeneity of ECM signals and is not recapitulated by
static and uniform hydrogel systems. However, the complex combination of signals present in the cellular microenvironment may be mimicked by patterned hydrogel systems. Such advances allow
the introduction of spatially specific cues in hydrogels, making multicellular constructs, either through co-cultures or multilineage differentiation, a possibility16,17,18. Spatial
patterning of hydrogels also provides an additional tool for the development of high-throughput screening technology for the rapid investigation of cell–material interactions19,20,21,22.
High-throughput techniques may be particularly important as cell fate is simultaneously influenced by cell–cell interactions, soluble signals, cell–ECM interactions, cell shape and
mechanical forces, and natural ECMs often present cells with many distinct signals simultaneously23. Therefore, it is logical to expect that spatially patterned synthetic hydrogels will be
needed to effectively probe the simultaneous influence of many distinct signals in order to gain meaningful biological insights or to direct cellular behaviour. Novel chemistry, and the
application of orthogonal reactions to networks, now allows the unprecedented patterning of peptides and proteins or the alteration of network structure, even in the presence of cells. For
example, multiphoton processes were used to decorate biological moieties throughout 3D systems, relying on the coupling of acrylate-modified molecules in proteolytically degradable
polyethylene glycol (PEG) gels containing excess reactive groups24. A subsequent study illustrated the use of this technique to define regions of precise concentration and to pattern with
multiple molecules throughout PEG hydrogels25, further expanding their complexity. In another example, orthogonal binding pairs of barnase–barstar and streptavidin–biotin were applied
sequentially to pattern multiple growth factors and biological molecules (Fig. 2)26. Specifically, one component of the pair was first immobilized into the hydrogel using two-photon
chemistry and the corresponding binding partner was subsequently introduced. By applying different binding pairs in sequence, multiple factors could be immobilized. This approach follows a
previously developed method where a tethered photolabile moiety was illuminated to introduce cell adhesion molecules and guide cell migration27. Light has also been used to introduce
patterned channels that permit the migration of cells through a hydrogel28. Other systems have been developed where orthogonal and cytocompatible click chemistry can be used to first form,
and then pattern, hydrogels29. For example, copper-free click chemistry was used to form hydrogels around cells using the reaction of a PEG tetra-azide and a bis(DIFO3) di-functionalized
polypeptide (containing a proteolytic degradable group), then an orthogonal thiol-ene photocoupling reaction was used to modify the gels with a peptide. The sequential reactions and spatial
patterning permitted the gels to be controllably remodelled by cells (Fig. 2). A subsequent study combined the click chemistry with a photocleavable functionality, to fabricate spatially
patterned hydrogels and then to enable 3D cleavage of crosslinks30. Finally, a sequential crosslinking process31 was used to pattern cell spreading and remodelling by first encapsulating
cells in a hydrogel permissive to cell remodelling and then by introducing photo-induced crosslinks. The cells were unable to completely degrade the network, leading to changes in local cell
spreading and subsequent stem cell differentiation. HYDROGELS THAT DEGRADE WITH TIME Although stable and patterned hydrogels have been very useful as a means of studying fundamental
cell–material interactions, they over-simplify the complexity of the temporal dynamics present during both tissue development and repair processes. Specifically, the regulatory cascades
during tissue formation and repair are extremely complex and while ‘static’ hydrogels feature some dynamic components (for example, reversible receptor–ligand binding and dynamic cell–cell
interactions), little has been done to intentionally manipulate temporal properties in hydrogels, potentially owing to a lack of specific enabling technologies. However, the intrinsic
properties of hydrogels lend themselves to dynamic physical and biochemical applications, including responsiveness to changes in the signalling environment. Therefore, the interface between
dynamic biological systems and hydrogel material science provides an exciting area for future hydrogel design. The simplest approach to introduce temporal changes in hydrogels has been _via_
control of hydrogel degradation, primarily to release growth factors and to eliminate the permanent implantation of a material32. One of the earliest examples of a degradable hydrogel
features chains of poly(α-hydroxy esters), such as poly(lactic acid), incorporated at the ends of PEG molecules before the introduction of reactive groups for crosslinking33. Other examples
include materials based on poly(vinyl alcohol)34, poly(propylene fumarates)35, dextrans36 and hyaluronic acid37. In all examples, the crosslinking material changes with time, altering the
physical properties and diffusivity and eventually completely degrading away (Fig. 3). Likewise, non-covalently crosslinked hydrogels (for example, _via_ ionic interactions), such as
alginate, can be manipulated to alter dissociation kinetics through changes in polymer chemistry38. One area in which degradation has been particularly useful is in controlling the
distribution of ECM molecules by encapsulated cells37. In tissue engineering, it is important that these molecules are well distributed, to build up a tissue that can replicate both the form
and function of native tissues. In cartilage tissue engineering approaches (with both PEG39 and hyaluronic acid37 hydrogels), the inclusion of fractions of hydrolytically degradable
components has improved this distribution and led to improved properties, particularly functional mechanical properties. Likewise, simple hydrolytic degradation mechanisms have also hinted
at the concept of dynamically influencing tissue formation _via_ local release of ‘morphogens’ 40,41. The adaptability of hydrogel synthesis allows one to introduce signalling molecules
_via_ covalent linkage, non-covalent tethering or physical entrapment42, or as localized depots40,41,43, leading to spatial morphogen gradients that mimic a common paradigm in tissue
development and regeneration. Non-covalent tethering in a hydrogel can be used as a mechanism to control diffusivity of general classes of growth factors such as heparin binders44, or
specific growth factors such as nerve growth factor45. In an alternative mechanism, hydrogels are also designed to be susceptible to degradation by the proteases used by cells to remodel
their surroundings; specifically, peptides that may be cleaved by cell-produced proteases are incorporated into the hydrogel crosslinks46,47. A wide range of sequences were investigated that
are degraded by matrix metalloproteinases (MMPs), elastases and plasmin2,48,49. The general susceptibility to proteases is controlled by the specific peptide sequence and there are a
plethora of sequences that could be used to tailor the specific cell-mediated degradation of hydrogels (Fig. 3). Generally, the hydrogels are formed by reacting a multifunctional polymer
(for example, PEG macromers with vinyl sulphones or acrylates) with end groups of protease-sensitive peptides (for example, thiols from cysteine moieties), where cells and molecules can be
encapsulated during gelation. The remodelling of hydrogels by cells was observed for hydrogels where both sequences for adhesion and degradation were present50, with degradation rates
controlled by crosslink density and peptide specificity51. This approach has been used in numerous cases to permit cell-mediated degradation of hydrogels, leading to engineered constructs
for tissues such as bone and vascular structures49,52, including examples of the incorporation of growth factors released _via_ cellular cues53. Likewise, these matrices are useful as cell
carriers that permit cellular morphogenesis into a variety of tissue structures46,54. Hybrid systems of synthetic and biological polymers including PEG fibrinogen28 and hyaluronic acid55
have also been developed with similar functionality. This approach can also be harnessed to control the delivery of molecules in diseases where protease activity is altered. Some examples of
this include rheumatoid arthritis56, cancer57 and after myocardial infarction58. In these cases, MMP levels deviate from equilibrium after injury. Thus, a system that exhibits MMP
sensitivity may degrade differently depending on local MMP levels. This provides a feedback approach where local molecule delivery is regulated by cellular activity, increasing the available
intricacy of release profiles. Likewise, MMP-sensitive tethers have been used for release that correlates with increased MMP activity59,60. In these cases, a pendant drug is released from
matrices in response to local protease levels. These examples represent an initial foray into biological responsiveness, in the sense that hydrogels change their properties in response to a
specific set of biological stimuli. Beyond purely synthetic materials, the development of protein-based materials is also becoming a possibility. In this case, molecular engineering allows
for the precise introduction of a range of signal response mechanisms to control hydrogel properties (for example, _via_ crosslinking) or cellular interactions (for example, _via_
degradation sites)61. This area is likely to expand in upcoming years, as the need for the complexity found in native tissues is realized. Approaches such as directed evolution will allow
the rapid assessment of sequences with specific functionality. As an alternative to covalently crosslinked structures, hydrogels may be formed _via_ the self-assembly of peptides designed to
form fibrillar structures or even to present biological signals6. The same cues for adhesion and degradation can be incorporated into such structures62, and because self-assembling
hydrogels are amenable to modular design, it is possible to optimize these hydrogels for a particular outcome such as endothelialization63. Proteins can also be specifically engineered for
added functionality and cooperativity with integrin-mediated adhesion64 or for binding to specific molecules5. Finally, spatial control, as described in the previous section, may also be
introduced in a dynamic fashion, providing spatiotemporal control of many hydrogel features and adding increased complexity. TRIGGERED CHANGES IN HYDROGEL PROPERTIES The use of hydrogels
with either user-controlled degradation or crosslinking, or biologically responsive feedback mechanisms, highlights many novel advances in the field of hydrogel design. Moving with
complexity, the field of hydrogel design is advancing past systems that change with time owing to simple hydrolysis or through cell-mediated proteolysis. Although these systems have advanced
our understanding of dynamic cell–material interactions, they cannot replicate the temporal changes that occur throughout development or permit one to investigate the timed effects of
specific cues on cell function. The most commonly used dynamic hydrogels to date have been designed to respond to changes in temperature and pH. For example, hydrogels composed of
poly(_N_-isopropyl acrylamide) and poly(acrylic acid) derivatives can undergo substantial changes in volume, shape, mesh size, mechanical stiffness and optical transparency in response to
temperature and/or pH. Hydrogels that include temperature-responsive or pH-responsive polymers have also been shown to dynamically vary ligand presentation to cells65,66,67, and to trigger
the release of cells and cell sheets for tissue engineering applications68. These technologies have enabled proof-of-concept for new drug delivery and tissue engineering strategies; however,
changes in environmental pH, temperature or ionic strength may be detrimental in some tissue engineering applications and in biological systems that typically exist under regulated
homeostatic conditions. Alternatively, light is able to present precise control over temporal and spatial signals. Photoinitiated polymerizations have been widely used to form hydrogels for
cell encapsulation and for the patterning of biological signals within hydrogels69. Light has recently been used as a trigger for the breaking of crosslinks in hydrogel networks, namely
through the introduction of photosensitive _o_-nitro benzyl groups into the crosslinks70 (Fig. 4). This approach led to control over hydrogel structure in space and time, as well as the
release of tethered signals. This synthetic system was used to probe how dynamic mechanical properties influence the phenotype of valvular interstitial cells, where changes in mechanical
properties led to alterations in cell phenotype71. This material platform is relevant in a wide range of studies where dynamic properties are desired and is likely to push the boundaries of
tunable systems. Beyond degradation, there are many physiological processes where an increase in crosslinking may be of interest. For example, tissues experience increased mechanics during
development and in various disease states, such as scar formation and tumour development72. There are only a few examples of systems where stiffness can be dynamically increased.
Collagen-alginate composite hydrogels have been investigated, demonstrating mechanical properties altered by the introduction of divalent cations73. It is important to note that the
introduction of calcium may also alter cell signalling. External stimuli such as pH can also be used to alter matrix stiffness74; however, these approaches may also lead to other undesirable
changes in properties such as hydrophobicity or swelling. Another class of materials involves DNA crosslinked hydrogels where the presence of free DNA may lead to increased stiffening75,76,
with networks that change over a period of hours. Sequential crosslinking systems can also be used to increase hydrogel crosslinking by applying two modes of crosslinking in series2,
varying crosslink density and in some cases susceptibility to cell-mediated degradation (Fig. 4). This was recently used to probe the timing of mechanical changes on mesenchymal stem cell
fate77. As a final example, the kinetics of gelation (crosslinking of thiolated hyaluronic acid with PEG diacrylate, Fig. 4) has been exploited to temporally alter substrate mechanics,
mimicking changes that occur during the maturation of cardiomyocytes from mesoderm to adult myocardium78. Although the majority of studies to date have focused on hydrogels that respond to
physicochemical stimuli (that is, light, heat and pH), emerging concepts are also focusing on hydrogels that respond to specific biological stimuli, such as protease-cleavable crosslinks
within hydrogels. Beyond this, ‘biologically inspired’ mechanisms such as enzyme catalysis79, competitive ligand–receptor binding80 and even nanometer-scale protein motions81 may also be
used to trigger changes in hydrogel properties (Fig. 5). In some cases, these hydrogels can respond to a biological input by releasing an output, as demonstrated by insulin release in
response to glucose catalysis82 or biochemically triggered growth factor release83,84. The full potential of bioresponsive materials is becoming apparent, and is likely to become clearer in
the coming decade. Bioresponsiveness may ultimately enable investigators to mimic key functions of secretory organs in the body, such as the pancreas. Importantly, they may also allow
biologists and bioengineers to address new hypotheses in cell and developmental biology. Next-generation hydrogels may be designed to respond to cell-secreted biological inputs, in a manner
that mimics, or even actively manipulates, the dynamics of tissue development and regeneration. Bioresponsiveness could be particularly relevant in studying and manipulating ‘emergent’
processes, such as stem cell differentiation, in which hydrogel properties that are appropriate in the early stages are likely to be dramatically different from those needed later on. For
example, recent studies in standard cell culture indicate that human embryonic stem cell differentiation into spinal motor neurons85 or cardiomyocytes86 can be amplified by mimicking the
timed soluble signalling regimen observed during early tissue development, and that the timing of signal delivery is critical. One can envision a more intelligent embodiment of this
approach, in which a hydrogel responds to a change in stem cell phenotype (for example, initial lineage commitment) by changing local physical properties or by delivering a specific growth
factor, thereby optimizing new tissue formation. We have only begun to develop the type of bioresponsiveness needed to manipulate tissue development in this way, and innovative synthetic
strategies are critically important to expand this new area. HYDROGELS THAT MANIPULATE TISSUE FORMATION Tissue formation processes include complex and interdependent changes in diverse
environmental parameters, notably cell–ECM adhesion, cell-mediated ECM remodelling, cell–cell interaction, cell migration, soluble signalling and ECM mechanics. The complex dynamics of
natural cell–ECM interactions provide both a challenge and a template for hydrogel design. Some emerging technologies in hydrogel design relate to bioinspired regulation of tissue formation,
such as mimicking the ability of natural ECMs to sequester endogenous, cell-secreted signals. Sequestering can be used as a mechanism to downregulate endogenous signals that negatively
impact tissue healing, such as tumour necrosis factor α (ref. 87). In other circumstances, sequestering may result in local amplification of signals that promote healing by ‘harnessing’
endogenous, cell-secreted growth factors6,88,89. Harnessing of endogenous factors may lead to a series of practical advantages when compared with delivery of purified recombinant factors.
These include enhanced biological activity of native, post-translationally modified molecules, simplified paths to clinical regulatory approval and the possibility of manipulating cell–cell
paracrine signalling. Interestingly, sequestering and harnessing mechanisms are also dependent on the ever-changing characteristics of the local environment, such as the concentration of
cell-secreted growth factors. Therefore, hydrogels that sequester signalling molecules may soon be designed to evolve with time to optimally regulate new tissue formation. For example, it
may be possible to sequester particular cytokines or growth factors during early wound healing, and then release them when needed during later stages of tissue formation. These
time-dependent and environment-dependent regulatory mechanisms are common during natural wound healing, but have yet to be exploited in engineered hydrogels. Thus, as the last decade has
included major advances in our ability to design complex systems, the next decade is likely to push these systems towards the complexity of biological processes and perhaps exceeding their
level of control. In principle, many contemporary hydrogel synthesis schemes combine multiple dynamic, bioresponsive mechanisms into a single hydrogel. For example, glucose catalysis has led
to pH-dependent hydrogel swelling and associated insulin release90, while protein conformational changes have led to bioresponsive changes in hydrogel swelling and associated growth factor
release. The combination of these triggers would lead to unprecedented control of bioresponsiveness and molecule delivery for a specific application. The field has only begun to explore the
design space of potential combinations, and the diversity of natural ECMs suggests that a wide range of dynamic functions are possible and perhaps required to achieve functional tissue
engineering. MOVING FORWARD IN HYDROGEL DESIGN In the future, we may see generic synthetic strategies to produce hydrogels that respond predictably to virtually any desired input. These
systems may be used to achieve independent, dynamic regulation of multiple parameters during tissue formation and avoid the confounding effects of lurking variables, potentially _via_ both
advanced material design and high-throughput synthesis and characterization technologies. The structure of these materials may also evolve as hydrogels mimic many aspects of biological
tissues, but rarely possess the hierarchical structure (for example, fibres) that provides organization and points of contact for cells. Advances in hydrogel design over the upcoming years
will be made by collaboration between material scientists, bioengineers and cell biologists, and time will tell where the true utility of such advanced hydrogel systems is found. Biological
complexity is partly due to cellular interactions and the dynamic nature of the local cell microenvironments, and it is not entirely clear what the level of complexity should be in the
interacting hydrogel. Finally, the majority of the examples described here focused on _in vitro_ analysis, with only a few examples of implanted hydrogels. Thus, the added complexity found
_in vivo_ needs to be addressed in the context of such materials systems. However, it is clear that such systems will allow the pursuit of questions that were unanswerable with simple static
material systems and will lead to new approaches for the treatment of damaged tissues. ADDITIONAL INFORMATION HOW TO CITE THIS ARTICLE: Burdick J.A. and Murphy W.L. Moving from static to
dynamic complexity in hydrogel design. _Nat. Commun._ 3:1269 doi: 10.1038/ncomms2271 (2012). REFERENCES * Jia X. Q. & Kiick K. L. Hybrid multicomponent hydrogels for tissue engineering.
_Macromol. Biosci._ 9, 140–156 (2009). Article CAS PubMed PubMed Central Google Scholar * Khetan S., Katz J. S. & Burdick J. A. Sequential crosslinking to control cellular spreading
in 3-dimensional hydrogels. _Soft Matter_ 5, 1601–1606 (2009). Article ADS CAS Google Scholar * Sands R. W. & Mooney D. J. Polymers to direct cell fate by controlling the
microenvironment. _Curr. Opin. Biotechnol._ 18, 448–453 (2007). Article CAS PubMed PubMed Central Google Scholar * Huebsch N. et al. Harnessing traction-mediated manipulation of the
cell/matrix interface to control stem-cell fate. _Nat. Mater._ 9, 518–526 (2010).) _DEMONSTRATED THAT MATRIX STIFFNESS REGULATES THE CELL'S NANOSCALE REORGANIZATION OF ADHESION LIGANDS,
WHICH INFLUENCES STEM CELL DIFFERENTIATION_. Article ADS CAS PubMed PubMed Central Google Scholar * Sakiyama-Elbert S. E. & Hubbell J. A. Controlled release of nerve growth factor
from a heparin-containing fibrin-based cell ingrowth matrix. _J. Control Release_ 69, 149–158 (2000). Article CAS PubMed Google Scholar * Shah R. N. et al. Supramolecular design of
self-assembling nanofibers for cartilage regeneration. _Proc. Natl Acad. Sci. USA_ 107, 3293–3298 (2010). Article ADS CAS PubMed PubMed Central Google Scholar * Wood M. D. et al.
Affinity-based release of glial-derived neurotrophic factor from fibrin matrices enhances sciatic nerve regeneration. _Acta Biomater._ 5, 959–968 (2009). Article CAS PubMed Google Scholar
* Discher D. E., Mooney D. J. & Zandstra P. W. Growth factors, matrices, and forces combine and control stem cells. _Science_ 324, 1673–1677 (2009). Article ADS CAS PubMed PubMed
Central Google Scholar * Mann C. & Leckband D. Measuring traction forces in long-term cell cultures. _Cell Mol. Bioeng._ 3, 40–49 (2010). Article CAS Google Scholar * Wang Y. L.
& Pelham R. J. Preparation of a flexible, porous polyacrylamide substrate for mechanical studies of cultured cells. _Method Enzymol._ 298, 489–496 (1998). Article CAS Google Scholar *
Engler A. J., Sen S., Sweeney H. L. & Discher D. E. Matrix elasticity directs stem cell lineage specification. _Cell_ 126, 677–689 (2006). Article CAS PubMed Google Scholar *
Gilbert P. M. et al. Substrate elasticity regulates skeletal muscle stem cell self-renewal in culture. _Science_ 329, 1078–1081 (2010). Article ADS CAS PubMed PubMed Central Google
Scholar * Saha K. et al. Substrate modulus directs neural stem cell behavior. _Biophys. J._ 95, 4426–4438 (2008). Article ADS CAS PubMed PubMed Central Google Scholar * Shin J. W.,
Swift J., Spinler K. R. & Discher D. E. Myosin-II inhibition and soft 2D matrix maximize multinucleation and cellular projections typical of platelet-producing megakaryocytes. _Proc.
Natl Acad. Sci. USA_ 108, 11458–11463 (2011). Article ADS CAS PubMed PubMed Central Google Scholar * Slaughter B. V., Khurshid S. S., Fisher O. Z., Khademhosseini A. & Peppas N. A.
Hydrogels in regenerative medicine. _Adv. Mater._ 21, 3307–3329 (2009). Article CAS PubMed PubMed Central Google Scholar * Koh W. G., Itle L. J. & Pishko M. V. Molding of hydrogel
microstructures to create multiphenotype cell microarrays. _Anal. Chem._ 75, 5783–5789 (2003). Article CAS PubMed Google Scholar * Ling Y. et al. A cell-laden microfluidic hydrogel.
_Lab. Chip_ 7, 756–762 (2007). Article CAS PubMed Google Scholar * Albrecht D. R., Underhill G. H., Wassermann T. B., Sah R. L. & Bhatia S. N. Probing the role of multicellular
organization in three-dimensional microenvironments. _Nat. Methods_ 3, 369–375 (2006).) _USED DIELECTROPHORETIC FORCES IN HYDROGELS TO ORGANIZE THOUSANDS OF MULTICELLULAR CLUSTERS, IN WHICH
THE TISSUE ORGANIZATION INFLUENCED CHONDROCYTE FUNCTION_. Article CAS PubMed Google Scholar * King W. J., Jongpaiboonkit L. & Murphy W. L. Influence of FGF2 and PEG hydrogel matrix
properties on hMSC viability and spreading. _J. Biomed. Mater Res. A_ 93, 1110–1123 (2010). PubMed PubMed Central Google Scholar * Levengood S. L. & Murphy W. L. Biomaterials for
high-throughput stem cell culture. _Curr. Stem Cell Res. Ther._ 5, 261–267 (2010). Article CAS PubMed Google Scholar * Peters A., Brey D. M. & Burdick J. A. High-throughput and
combinatorial technologies for tissue engineering applications. _Tissue Eng. Part B Rev._ 15, 225–239 (2009). Article CAS PubMed Google Scholar * Gobaa S. et al. Artificial niche
microarrays for probing single stem cell fate in high throughput. _Nat. Methods_ 8, 949–955 (2011).) _USED HIGH THROUGHPUT ROBOTIC MICROSPOTTING TO EFFICIENTLY PROBE STEM CELL
DIFFERENTIATION AND IDENTIFY ARTIFICIAL NICHES FOR NEURAL STEM CELLS_. Article CAS PubMed Google Scholar * Hughes C. S., Postovit L. M. & Lajoie G. A. Matrigel: a complex protein
mixture required for optimal growth of cell culture. _Proteomics_ 10, 1886–1890 (2010). Article CAS PubMed Google Scholar * Lee S. H., Moon J. J. & West J. L. Three-dimensional
micropatterning of bioactive hydrogels via two-photon laser scanning photolithography for guided 3D cell migration. _Biomaterials_ 29, 2962–2968 (2008). Article CAS PubMed PubMed Central
Google Scholar * Hoffman J. C. & West J. L. Three-dimensional photolithographic patterning of multiple bioactive ligands in poly(ethylene glycol) hydrogels. _Soft Matter_ 6, 5056–5063
(2010). Article ADS CAS Google Scholar * Wylie R. G. et al. Spatially controlled simultaneous patterning of multiple growth factors in three-dimensional hydrogels. _Nat. Mater._ 10,
799–806 (2011). Article ADS CAS PubMed Google Scholar * Luo Y. & Shoichet M. S. A photolabile hydrogel for guided three-dimensional cell growth and migration. _Nat. Mater._ 3,
249–253 (2004). Article ADS CAS PubMed Google Scholar * Sarig-Nadir O., Livnat N., Zajdman R., Shoham S. & Seliktar D. Laser photoablation of guidance microchannels into hydrogels
directs cell growth in three dimensions. _Biophys. J._ 96, 4743–4752 (2009). Article ADS CAS PubMed PubMed Central Google Scholar * DeForest C. A., Polizzotti B. D. & Anseth K. S.
Sequential click reactions for synthesizing and patterning three-dimensional cell microenvironments. _Nat. Mater._ 8, 659–664 (2009). Article ADS CAS PubMed PubMed Central Google
Scholar * DeForest C. A. & Anseth K. S. Cytocompatible click-based hydrogels with dynamically tunable properties through orthogonal photoconjugation and photocleavage reactions. _Nat.
Chem._ 3, 925–931 (2011). _USED CLICK CHEMISTRY TO ACHIEVE ORTHOGONAL AND DYNAMIC CONTROL OF HYDROGEL PROPERTIES, WHICH ENABLED ORTHOGONAL STEM CELL ENTRAPMENT AND RECOVERY_. Article CAS
PubMed PubMed Central Google Scholar * Khetan S. & Burdick J. A. Patterning network structure to spatially control cellular remodeling and stem cell fate within 3-dimensional
hydrogels. _Biomaterials_ 31, 8228–8234 (2010). Article CAS PubMed Google Scholar * Anseth K. S. et al. _In situ_ forming degradable networks and their application in tissue engineering
and drug delivery. _J. Control. Release_ 78, 199–209 (2002). Article CAS PubMed Google Scholar * Metters A. T., Anseth K. S. & Bowman C. N. Fundamental studies of a novel,
biodegradable PEG-b-PLA hydrogel. _Polymer_ 41, 3993–4004 (2000). Article CAS Google Scholar * Martens P., Holland T. & Anseth K. S. Synthesis and characterization of degradable
hydrogels formed from acrylate modified poly(vinyl alcohol) macromers. _Polymer_ 43, 6093–6100 (2002). Article CAS Google Scholar * Jo S., Shin H. & Mikos A. G. Modification of
oligo(poly(ethylene glycol) fumarate) macromer with a GRGD peptide for the preparation of functionalized polymer networks. _Biomacromolecules_ 2, 255–261 (2001). Article ADS CAS PubMed
Google Scholar * De Groot C. J. et al. _In vitro_ biocompatibility of biodegradable dextran-based hydrogels tested with human fibroblasts. _Biomaterials_ 22, 1197–1203 (2001). Article CAS
PubMed Google Scholar * Sahoo S., Chung C., Khetan S. & Burdick J. A. Hydrolytically degradable hyaluronic acid hydrogels with controlled temporal structures. _Biomacromolecules_ 9,
1088–1092 (2008). Article CAS PubMed PubMed Central Google Scholar * Boontheekul T., Kong H. J. & Mooney D. J. Controlling alginate gel degradation utilizing partial oxidation and
bimodal molecular weight distribution. _Biomaterials_ 26, 2455–2465 (2005). Article CAS PubMed Google Scholar * Bryant S. J. & Anseth K. S. Controlling the spatial distribution of
ECM components in degradable PEG hydrogels for tissue engineering cartilage. _J. Biomed. Mater. Res. A_ 64A, 70–79 (2003). Article CAS Google Scholar * Nguyen E. H., Schwartz M. P. &
Murphy W. L. Biomimetic approaches to control soluble concentration gradients in biomaterials. _Macromol. Biosci._ 11, 483–492 (2011). Article CAS PubMed PubMed Central Google Scholar *
Singh M., Berkland C. & Detamore M. S. Strategies and applications for incorporating physical and chemical signal gradients in tissue engineering. _Tissue Eng. Part B Rev._ 14, 341–366
(2008). Article CAS PubMed PubMed Central Google Scholar * Hudalla G. A. & Murphy W. L. Biomaterials that regulate growth factor activity via bioinspired interactions. _Adv. Funct.
Mater._ 21, 1754–1768 (2011). Article CAS PubMed PubMed Central Google Scholar * Peret B. J. & Murphy W. L. Controllable soluble protein concentration gradients in hydrogel
networks. _Adv. Funct. Mater._ 18, 3410–3417 (2008). Article CAS PubMed PubMed Central Google Scholar * Nie T., Baldwin A., Yamaguchi N. & Kiick K. L. Production of
heparin-functionalized hydrogels for the development of responsive and controlled growth factor delivery systems. _J. Control. Release_ 122, 287–296 (2007). Article CAS PubMed PubMed
Central Google Scholar * Lee A. C. et al. Controlled release of nerve growth factor enhances sciatic nerve regeneration. _Exp. Neurol._ 184, 295–303 (2003). Article CAS PubMed Google
Scholar * Kraehenbuehl T. P. et al. Three-dimensional extracellular matrix-directed cardioprogenitor differentiation: systematic modulation of a synthetic cell-responsive PEG-hydrogel.
_Biomaterials_ 29, 2757–2766 (2008). Article CAS PubMed Google Scholar * Lutolf M. P. et al. Repair of bone defects using synthetic mimetics of collagenous extracellular matrices. _Nat.
Biotechnol._ 21, 513–518 (2003). Article CAS PubMed Google Scholar * Gobin A. S. & West J. L. Cell migration through defined, synthetic extracellular matrix analogues. _FASEB J._ 16,
751–753 (2002). Article CAS PubMed Google Scholar * Lutolf M. P. et al. Synthetic matrix metalloproteinase-sensitive hydrogels for the conduction of tissue regeneration: engineering
cell-invasion characteristics. _Proc. Natl Acad. Sci. USA_ 100, 5413–5418 (2003). Article ADS CAS PubMed PubMed Central Google Scholar * Lutolf M. P., Raeber G. P., Zisch A. H.,
Tirelli N. & Hubbell J. A. Cell-responsive synthetic hydrogels. _Adv. Mater._ 15, 888–892 (2003). Article CAS Google Scholar * Patterson J. & Hubbell J. A. Enhanced proteolytic
degradation of molecularly engineered PEG hydrogels in response to MMP-1 and MMP-2. _Biomaterials_ 31, 7836–7845 (2010). Article CAS PubMed Google Scholar * Seliktar D., Zisch A. H.,
Lutolf M. P., Wrana J. L. & Hubbell J. A. MMP-2 sensitive, VEGF-bearing bioactive hydrogels for promotion of vascular healing. _J. Biomed. Mater. Res. A_ 68A, 704–716 (2004). Article
CAS Google Scholar * Sakiyama-Elbert S. E., Panitch A. & Hubbell J. A. Development of growth factor fusion proteins for cell-triggered drug delivery. _FASEB J._ 15, 1300–1302 (2001).
Article CAS PubMed Google Scholar * Hanjaya-Putra D. et al. Controlled activation of morphogenesis to generate a functional human microvasculature in a synthetic matrix. _Blood_ 118,
804–815 (2011). Article CAS PubMed PubMed Central Google Scholar * Burdick J. A. & Prestwich G. D. Hyaluronic acid hydrogels for biomedical applications. _Adv. Mater._ 23, H41–H56
(2011). Article CAS PubMed PubMed Central Google Scholar * Burrage P. S., Mix K. S. & Brinckerhoff C. E. Matrix metalloproteinases: role in arthritis. _Front. Biosci._ 11, 529–543
(2006). Article CAS PubMed Google Scholar * Kerkela E. & Saarialho-Kere U. Matrix metalloproteinases in tumor progression: focus on basal and squamous cell skin cancer. _Exp.
Dermatol._ 12, 109–125 (2003). Article CAS PubMed Google Scholar * Spinale F. G. Myocardial matrix remodeling and the matrix metalloproteinases: influence on cardiac form and function.
_Physiol. Rev._ 87, 1285–1342 (2007). Article CAS PubMed Google Scholar * Kim H. S. & Yoo H. S. MMPs-responsive release of DNA from electrospun nanofibrous matrix for local gene
therapy: _in vitro_ and _in vivo_ evaluation. _J. Control. Release_ 145, 264–271 (2010). Article CAS PubMed Google Scholar * Tauro J. R. & Gemeinhart R. A. Matrix metalloprotease
triggered delivery of cancer chemotherapeutics from hydrogel matrixes. _Bioconjug. Chem._ 16, 1133–1139 (2005). Article CAS PubMed Google Scholar * Wong Po Foo C. T., Lee J. S.,
Mulyasasmita W., Parisi-Amon A. & Heilshorn S.C. Two-component protein-engineered physical hydrogels for cell encapsulation. _Proc. Natl Acad. Sci. USA_ 106, 22067–22072 (2009). Article
ADS PubMed PubMed Central Google Scholar * Sengupta D. & Heilshorn S. C. Protein-engineered biomaterials: highly tunable tissue engineering scaffolds. _Tissue Eng. Part B-Rev._ 16,
285–293 (2010). Article CAS PubMed Google Scholar * Jung J. P., Moyano J. V. & Collier J. H. Multifactorial optimization of endothelial cell growth using modular synthetic
extracellular matrices. _Integr. Biol._ 3, 185–196 (2011). _MODULAR, SELF-ASSEMBLING PEPTIDES FORMED HIGHLY ADAPTABLE HYDROGELS, WHICH ENABLED EFFICIENT MULTIFACTORIAL OPTIMIZATION OF CELL
BEHAVIOR_. Article CAS Google Scholar * Martino M. M. et al. Engineering the growth factor microenvironment with fibronectin domains to promote wound and bone tissue healing. _Sci.
Transl. Med._ 3, 100ra189 (2011). Article ADS CAS Google Scholar * Ebara M. et al. The effect of extensible PEG tethers on shielding between grafted thermo-responsive polymer chains and
integrin-RGD binding. _Biomaterials_ 29, 3650–3655 (2008). Article CAS PubMed Google Scholar * Hoffman A. S. & Stayton P. S. Conjugates of stimuli-responsive polymers and proteins.
_Prog. Polym. Sci._ 32, 922–932 (2007). Article CAS Google Scholar * Hoffman A. S. et al. Conjugates of stimuli-responsive polymers and biomolecules: random and site-specific conjugates
of temperature-sensitive polymers and proteins. _Macromol. Symp._ 118, 553–563 (1997). Article CAS Google Scholar * Ebara M. et al. Copolymerization of 2-carboxyisopropylacrylamide with
N-isopropylacrylamide accelerates cell detachment from grafted surfaces by reducing temperature. _Biomacromolecules_ 4, 344–349 (2003). Article CAS PubMed Google Scholar * Ifkovits J. L.
& Burdick J. A. Review: photopolymerizable and degradable biomaterials for tissue engineering applications. _Tissue Eng._ 13, 2369–2385 (2007). Article CAS PubMed Google Scholar *
Kloxin A. M., Kasko A. M., Salinas C. N. & Anseth K. S. Photodegradable hydrogels for dynamic tuning of physical and chemical properties. _Science_ 324, 59–63 (2009). _PHOTODEGRADABLE
HYDROGELS ENABLED REAL-TIME, "USER-DEFINED" MANIPULATION OF MATERIAL PROPERTIES AND, IN TURN, CHONDROGENIC DIFFERENTIATION OF STEM CELLS_. Article ADS CAS PubMed PubMed Central
Google Scholar * Kloxin A. M., Benton J. A. & Anseth K. S. _In situ_ elasticity modulation with dynamic substrates to direct cell phenotype. _Biomaterials_ 31, 1–8 (2010). Article
CAS PubMed Google Scholar * Paszek M. J. et al. Tensional homeostasis and the malignant phenotype. _Cancer Cell_ 8, 241–254 (2005). Article CAS PubMed Google Scholar * Gillette B. M.,
Jensen J. A., Wang M. X., Tchao J. & Sia S. K. Dynamic hydrogels: switching of 3D microenvironments using two-component naturally derived extracellular matrices. _Adv. Mater._ 22,
686–691 (2010). Article CAS PubMed Google Scholar * Yoshikawa H. Y. et al. Quantitative evaluation of mechanosensing of cells on dynamically tunable hydrogels. _JACS_ 133, 1367–1374
(2011). _TRIBLOCK COPOLYMER HYDROGELS WITH A PH-SENSITIVE COMPONENT WERE USED TO TUNE HYDROGEL ELASTICITY OVER A WIDE RANGE, RESULTING IN DYNAMIC CONTROL OVER MYOBLAST ADHESION AND
MORPHOLOGY_. Article CAS Google Scholar * Jiang F. X., Yurke B., Schloss R. S., Firestein B. L. & Langrana N. A. The relationship between fibroblast growth and the dynamic stiffnesses
of a DNA crosslinked hydrogel. _Biomaterials_ 31, 1199–1212 (2010). Article CAS PubMed Google Scholar * Jiang F. X., Yurke B., Schloss R. S., Firestein B. L. & Langrana N. A. Effect
of dynamic stiffness of the substrates on neurite outgrowth by using a DNA-crosslinked hydrogel. _Tissue Eng. Part A_ 16, 1873–1889 (2010). Article CAS PubMed Google Scholar *
Guvendiren M. & Burdick J. A. Stiffening hydrogels to probe short- and long-term cellular responses to dynamic mechanics. _Nat. Commun._ 3, 792 (2012).) _LIGHT-MEDIATED CROSSLINKING WAS
USED TO STIFFEN HYDROGELS IN THE PRESENCE OF CELLS, AND DYNAMIC STIFFENING MODULATED STEM CELL DIFFERENTIATION_. Article ADS PubMed CAS Google Scholar * Young J. L. & Engler A. J.
Hydrogels with time-dependent material properties enhance cardiomyocyte differentiation in vitro. _Biomaterials_ 32, 1002–1009 (2011). Article CAS PubMed Google Scholar * Ulijn R. V.
Enzyme-responsive materials: a new class of smart biomaterials. _J. Mater. Chem._ 16, 2217–2225 (2006). Article CAS Google Scholar * Miyata T., Asami N. & Uragami T. A reversibly
antigen-responsive hydrogel. _Nature_ 399, 766–769 (1999). Article ADS CAS PubMed Google Scholar * Murphy W. L. Emerging area: biomaterials that mimic and exploit protein motion. _Soft
Matter_ 7, 3679–3688 (2011). Article ADS CAS PubMed PubMed Central Google Scholar * Horbett T. A. & Klumb L. A. Design of insulin delivery devices based on glucose sensitive
membranes. _J. Control. Release._ 18, 59–80 (1992). Article Google Scholar * King W. J., Toepke M. W. & Murphy W. L. Facile formation of dynamic hydrogel microspheres for triggered
growth factor delivery. _Acta Biomater._ 7, 975–985 (2011). Article CAS PubMed Google Scholar * Sui Z., King W. J. & Murphy W. L. Dynamic materials based on a protein conformational
change. _Adv. Mater._ 19, 3377–3380 (2007). Article CAS Google Scholar * Li X. J. et al. Specification of motoneurons from human embryonic stem cells. _Nat. Biotechnol._ 23, 215–221
(2005). Article PubMed CAS Google Scholar * Laflamme M. A. et al. Cardiomyocytes derived from human embryonic stem cells in pro-survival factors enhance function of infarcted rat hearts.
_Nat. Biotechnol._ 25, 1015–1024 (2007). Article CAS PubMed Google Scholar * Lin C. C., Metters A. T. & Anseth K. S. Functional PEG-peptide hydrogels to modulate local inflammation
induced by the pro-inflammatory cytokine TNFalpha. _Biomaterials_ 30, 4907–4914 (2009). Article CAS PubMed PubMed Central Google Scholar * Hudalla G. A., Kouris N. A., Koepsel J. T.,
Ogle B. M. & Murphy W. L. Harnessing endogenous growth factor activity modulates stem cell behavior. _Integr. Biol. (Camb)_ 3, 832–842 (2011). _MOLECULAR SEQUESTERING LED TO AMPLIFIED
ENDOGENOUS GROWTH FACTOR SIGNALING AND INCREASED STEM CELL EXPANSION OR DIFFERENTIATION_. Article CAS Google Scholar * Levenstein M. E. et al. Secreted proteoglycans directly mediate
human embryonic stem cell-basic fibroblast growth factor 2 interactions critical for proliferation. _Stem Cells_ 26, 3099–3107 (2008). Article PubMed PubMed Central Google Scholar * Kost
J., Horbett T. A., Ratner B. D. & Singh M. Glucose-sensitive membranes containing glucose oxidase: activity, swelling, and permeability studies. _J. Biomed. Mater. Res._ 19, 1117–1133
(1985). Article CAS PubMed Google Scholar Download references AUTHOR INFORMATION AUTHORS AND AFFILIATIONS * Department of Bioengineering, University of Pennsylvania, 240 Skirkanich Hall,
210 S. 33rd street, Philadelphia, Pennsylvania 19104, USA, Jason A. Burdick * Departments of Biomedical Engineering and Orthopedics and Rehabilitation, University of Wisconsin, Madison,
53706, Wisconsin, USA William L. Murphy Authors * Jason A. Burdick View author publications You can also search for this author inPubMed Google Scholar * William L. Murphy View author
publications You can also search for this author inPubMed Google Scholar CORRESPONDING AUTHOR Correspondence to Jason A. Burdick. ETHICS DECLARATIONS COMPETING INTERESTS The authors declare
no competing financial interests. RIGHTS AND PERMISSIONS Reprints and permissions ABOUT THIS ARTICLE CITE THIS ARTICLE Burdick, J., Murphy, W. Moving from static to dynamic complexity in
hydrogel design. _Nat Commun_ 3, 1269 (2012). https://doi.org/10.1038/ncomms2271 Download citation * Received: 25 April 2012 * Accepted: 08 November 2012 * Published: 11 December 2012 * DOI:
https://doi.org/10.1038/ncomms2271 SHARE THIS ARTICLE Anyone you share the following link with will be able to read this content: Get shareable link Sorry, a shareable link is not currently
available for this article. Copy to clipboard Provided by the Springer Nature SharedIt content-sharing initiative