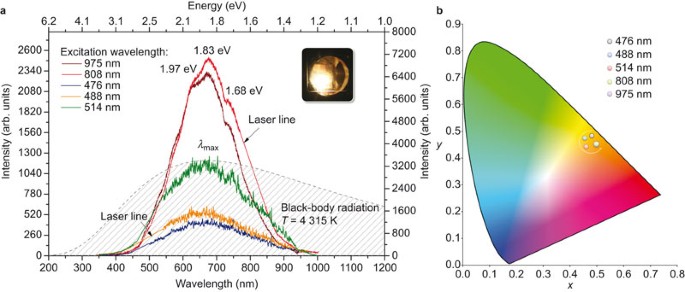
- Select a language for the TTS:
- UK English Female
- UK English Male
- US English Female
- US English Male
- Australian Female
- Australian Male
- Language selected: (auto detect) - EN
Play all audios:
ABSTRACT Recent theoretical and experimental studies have indicated the existence of a new stable phase of carbon with mixed sp2 and sp3 hybridized bonds—diaphite. Such a two-layered
structure with sp2/sp3 bonds may be observed after the photostimulation of highly oriented pyrolytic graphene with femtosecond laser pulses. This hidden multistability of graphene may be
used to create a semiconducting phase immersed in the semimetallic continuum, resulting in bandgap opening. We demonstrate that bandgap opening and light emission from graphene is possible
using continuous-wave laser beams with wavelengths from the visible (405 nm) to the near-infrared range (975 nm). We demonstrate that without the application of cooling, the effective
temperature of the emitting sample remains lower than 900 K, which is far below the value predicted by the theory of black-body radiation. Moreover, light emission from a graphene sample may
be observed at temperatures as low as 10 K. SIMILAR CONTENT BEING VIEWED BY OTHERS PLASMONICALLY ENHANCED MID-IR LIGHT SOURCE BASED ON TUNABLE SPECTRALLY AND DIRECTIONALLY SELECTIVE THERMAL
EMISSION FROM NANOPATTERNED GRAPHENE Article Open access 16 October 2020 ELECTROLUMINESCENCE AND ENERGY TRANSFER MEDIATED BY HYPERBOLIC POLARITONS Article 19 March 2025 DEEP-ULTRAVIOLET
ELECTROLUMINESCENCE AND PHOTOCURRENT GENERATION IN GRAPHENE/HBN/GRAPHENE HETEROSTRUCTURES Article Open access 08 December 2021 INTRODUCTION Because of its exceptional properties, graphene is
the focus of many extensive investigations. The two-dimensional crystal structure, linear energy dispersion and zero optical band gap of graphene, along with the presence of massless Dirac
quasiparticles, have successfully focused considerable scientific attention on this material, which theoretically should not exist.1 A number of studies have already reported many excellent
applications of graphene in various fields, e.g., new types of field-effect transistors,2 composite conductors3 and sensor applications.4,5 In addition to the interest in pristine graphene,
considerable scientific attention has also been devoted to the chemically derived hybrid known as graphene oxide, which exhibits unusual and tunable properties.6 As a chemical derivative,
graphene oxide possesses distinctly different properties than those of pristine graphene, which originate from the particular characteristics of the chemically reactive functional groups,
e.g., hydroxyl, epoxide, carbonyl and carboxyl groups, bound to the surface of grapheme.7 By controlling the sp2/sp3-hybridization ratio and the nature of the reactive groups, one is able to
control the optical and electrical properties of the resulting material.6 Recently, considerable scientific interest has also been devoted to studies of the white-light (WL) emission from
carbonaceous materials, using laser light or an electrical current as an excitation source. Incandescence experiments have been performed on a number of carbon structures, including carbon
nanotubes,8 fullerenes9,10 and graphene,11,12 which are being considered as materials for next-generation light sources. In particular, carbon nanotubes have been investigated for their
potential application as chemically stable thermal filaments in next-generation incandescent sources.13 However, although considerable efforts have been made in the development of
carbon-based devices,14 discussion of the WL emission from graphene is still severely lacking. It has previously been reported that pristine graphene exhibits no measurable light emission
under continuous-wave (CW) laser excitation; however, weak emission following the model of thermalized emission has been observed under femtosecond pulse excitation.15 The theory of
black-body radiation is naturally the first choice for the interpretation of the origin of light emissions from graphene and other carbonaceous materials.16 Various models have been proposed
to explain the origins of laser-induced incandescence, which is a complex phenomenon that involves laser absorption with the simultaneous emission of WL, which is responsible for cooling
the irradiated material.17 The observation of visible electroluminescence from multilayer graphene systems has also been reported by several authors.18 Biswas _et al_.19 have reported
tunable electroluminescence from planar graphene/SiO2 structures. Dong _et al_.20 have reported blue luminescence with a high quantum yield. Current-induced incandescent light emission has
also been observed in large-area graphene films by Yu and Dai.13 Recent studies of confined graphene systems have demonstrated the production of laser-excited WL emission from
edge-functionalized graphene quantum dots.21 To the best of our knowledge, we report here, for the first time, laser-induced WL emission from cooled (10 K) and moderate-temperature (<900
K) graphene ceramics. The purpose of this work was to investigate the WL emission from graphene ceramics with regard to the potential application of these materials as alternative WL
sources. The distinctive features of this WL emission include the independence of the emission spectra with respect to the ambient temperature between 10 K and 300 K; the spectral
independence of the emission band with respect to the excitation wavelength, which results in Stokes and anti-Stokes emission; the nonlinear dependence of the process; and the
wavelength-dependent power threshold. We demonstrate that without cooling, the effective temperature of the emitting sample remains lower than 900 K, which is far below the value predicted
by the theory of black-body radiation. Moreover, it is shown that laser-induced WL emission from graphene ceramics is also clearly observed at cryogenic temperatures as low as 10 K. The
results are discussed in terms of transient photoinduced domain-like phase conversion in disordered graphene systems. MATERIALS AND METHODS PREPARATION OF THE GRAPHENE CERAMICS The graphene
ceramics (GCs) were prepared from commercial (CheapTubes.com. Cambridgeport, VT, USA) graphene nanoplatelets. The graphene nanoplatelets were characterized by surface areas of 600–750 m2
g−1, the presence of 4–5 graphene layers with an average thickness of 8 nm, and a single-particle diameter of less than 2 μm (see Supplementary Information for transmission electron
microscopy images of the as-received graphene nanoplatelets). The GCs were fabricated in the form of pellets using the hot isostatic pressing method, which requires both an elevated
temperature and an isostatic pressure. As a first step, the graphene flakes were formed into pellets of 6 mm in diameter and 2 mm in thickness _via_ cold pressing at 0.2 GPa. The as-formed
pellets were then placed in a calcium carbonate (CaCO3) toroid and separated from the heating element by a thin layer of boron nitride. Each sample was then pressed at 8 GPa and heated to
500 °C for 1 min. As a final step, each GC sample was mechanically polished to remove the residual boron nitride powder and the topmost layers of graphene. Afterward, the sample was placed
in a vacuum chamber (see Supplementary Information for scanning electronic microscopy images of the GCs). SYNTHESIS OF LIYB0.99ER0.01P4O12 NANOTHERMOMETERS Erbium (Er3+)-doped lithium
ytterbium tetraphosphate nanocrystals were prepared _via_ a wet chemistry route. The starting materials for the synthesis were used without additional purification and were as follows:
(NH4)2HPO4 (POCH, 99.99%), erbium(III) oxide (Er2O3, 99.99%; Sigma-Aldrich, Poland), ytterbium(III) oxide (Yb2O3, 99.99%; Sigma-Aldrich) and Li2CO3 (POCH, 99.99%). Lanthanide ions were added
in the form of nitrates after prior dissolution of the corresponding oxides, e.g., Yb2O3 and Er2O3 were dissolved in concentrated nitric acid (HNO3). The (NH4)2HPO4 was dissolved in
deionized water and subsequently mixed with Li2CO3 and lanthanide nitrates containing appropriate amounts of Er3+ and Yb3+, resulting in a homogeneous solution. The LiYb0.99Er0.01P4O12
nanocrystals were obtained after the precipitation and transformation of the amorphous product into a crystalline product _via_ thermal treatment. The structural properties of the graphene
flakes were examined _via_ high-resolution transmission electron microscopy using a Philips CM20 SuperTwin transmission electron microscope, which provides a resolution of 0.25 nm at 200 kV
(see Supplementary Information). The luminescence spectra were measured using an Avantes AVS-USB2000 CCD spectrometer and a number of excitation laser sources, from the visible (VIS) up to
the near-infrared (NIR) regime: _λ_exc=975 nm, 808 nm, 514 nm, 488 nm, 476 nm and 405 nm. All graphene ceramics were measured in vacuum (10−3 hPa) and excited by a laser light focused to a
spot size of 1 mm. The temperature of the graphene ceramics was determined using LiYb0.99Er0.01P4O12 nanocrystals encrusted on the ceramic surface. The photoconductivity of the graphene
ceramics was measured _via_ the four-point method using four gold electrodes fixed with silver conducting paste. A 10-mA current source (Keithley 2002) was used in the experiment to measure
the voltage drop across the sample. RESULTS AND DISCUSSION The WL emission from the graphene ceramics was measured in oil-free vacuum (5×10−3 hPa). The samples were excited by a CW laser
beam focused to a spot of 1 mm in diameter. The laser-induced WL emission was investigated at a number of different excitation wavelengths, from the VIS up to the NIR range, i.e., at 476 nm,
488 nm, 514 nm, 808 nm and 975 nm. Representative spectra of the WL emission from the graphene ceramics upon irradiation at various excitation wavelengths are presented in Figure 1a. There
are several features of the data presented in Figure 1a that are worth noting. One distinctive feature of the data is its wide spectral range, extending over the VIS and NIR regions.
Notably, the emission occurs at energies higher than the energy of the exciting photons. This suggests that cascade multiphoton processes must be involved in this emission. At least three
characteristic maxima, at 1.97 eV (629 nm) and 1.83 eV (677 nm) and a shoulder at 1.68 eV (738 nm), may be identified in the emission band. It is worth noting that the presence of more than
one band maximum is in conflict with the theory of black-body radiation. A representative spectrum of black-body radiation for a temperature of _T_=4315 K is also presented for comparison.
No significant dependence of the broadband WL emission maxima on the excitation wavelengths was observed (Figure 1a). The peak maxima are located at nearly identical positions for each
wavelength, and only a slight broadening of the band is observed with decreasing excitation wavelength. The WL emission band is centered at approximately 1.87 eV (660 nm). The chromaticity
coordinates of the WL emission were calculated for all excitation wavelengths and are depicted in Figure 1b. Small shifts in the chromaticity coordinates were observed; however, all samples
were located in the white-orange region. Moreover, no particularly strong shifts in the emission-band maxima were observed for different excitation powers; however, it was observed that WL
emission from the graphene ceramics was a threshold process exhibiting supralinear behavior. The dependence of the laser-induced WL emission on the excitation light power is illustrated in
Figure 2. A representative plot of the WL emission excited at 975 nm for various laser powers is presented in Figure 2a. The nonlinear behavior of the excitation power, along with the
threshold point, is shown in Figure 2b for a number of excitation wavelengths. Once the threshold point is crossed, the WL emission increases in accordance with the power-law formula
(Equation (1)): where _I_WL is the intensity of the WL emission, _P_ is the power of the excitation laser light and _N_ is the order of the process. A monotonic dependence of the order of
the process on the excitation wavelength was observed (Figure 3). The lowest value of _N_=2.39 was observed for _λ_exc=405 nm, and the highest value of _N_=5.69 was observed for _λ_exc=975
nm. For high-energy photons (3.06 eV), the WL emission appears to behave like Stokes emission, whereas for lower-energy excitation, the emission may be treated as an anti-Stokes process.
This finding indicates that the WL emission from graphene ceramics has its origin in multiphoton absorption. The total energy _E_tot of _N_ absorbed photons with energy _ħ_ωexc was nearly
identical for all investigated excitation wavelengths, _E_tot=_N_exc_ħ_ωexc. If we take the photon energy corresponding to the _λ_exc=975 nm excitation wavelength (1.27 eV) and multiply it
by a number of photons equal to 5.69, we obtain a value of 7.2 eV, which is similar to the value calculated for _λ_exc=808 nm (1.53 eV), which yields 7.9 eV. The calculated values of _E_tot
for the wavelengths in the VIS region, i.e., 405 nm (3.06 eV), 476 nm (2.60 eV), 488 nm (2.54 eV) and 514 nm (2.41 eV), are 8.5 eV, 7.9 eV, 8.4 eV and 8.9 eV, respectively. The values of
_E_tot calculated for the VIS excitation photons are higher than those for the infrared photons, which may be related to the different index of refraction for and better focusing of the
visible light. The important observation is that regardless of the wavelength of the incident laser light used in the experiment, the total energy _E_tot of absorbed photons that gave rise
to the WL emission was comparable and seems to have remained constant across the entire measured region. This behavior may be well understood by considering the nearly frequency-independent
absorption of graphene, which, for pristine graphene, is equal to the universal value of _πα_ (2.29%) determined by the fine-structure constant _α_=_e__2__/ħc_. A careful analysis of the
power dependence of the WL emission revealed the threshold behavior of the WL emission. It was observed that _P_th decreased with increasing photon energy. The value of _P_th was at its
lowest for blue-region photons (see the inset of Figure 3). This threshold behavior is characteristic of the multiphoton absorption processes responsible for photon avalanching and
photoionization. Because the WL emission from the graphene ceramics was observed only under a reduced atmospheric pressure, it was necessary to investigate the impact of the pressure
conditions on the relative intensity of the WL emission. The results for the dependence on the ambient pressure are presented in Figure 4a. The intensity of the WL emission increased by a
factor of approximately five upon the reduction of the pressure by over five decades to 5×10−3 hPa (Figure 4b). Although this increase is quite significant, much higher values have been
observed in measurements of similar processes in lanthanide-doped dielectrics, e.g., Yb3+:YAG22 and LiYbP4O1223 nanocrystals. The dependence of the WL emission on the ambient pressure offers
some clue as to the origin of the emission, which cannot simply be attributed to the contribution of functional groups, as for the emission of graphene oxide. The observed supralinear
dependence of the intensity on the ambient pressure may be predicted by the model of the radiative dissipation of energy24 (Equation (2)): where _P_ is the measured pressure and _I_0 is the
initial intensity at pressure _P_0. It is clear that because of the observed saturation region, the results remain in agreement with this model; however, the lower dependence on the ambient
pressure suggests that the convection process does not impact the emission intensity of our graphene ceramics as strongly as is observed in the dielectrics Yb3+:YAG and LiYbP4O12. The theory
of black-body radiation is naturally the first choice for the interpretation of the origin of the WL emission in graphene. Although the nature of the photoluminescence bands is in conflict
with that of black-body radiation, the question of the effective temperature of the sample is still one of fundamental interest. As can be calculated based on Planck’s theorem and the
characteristics of the WL emission, the temperature of the sample is expected to be approximately 4300 K. Moreover, according to the theorem, a blue-shift of the emission band _λ_max should
be observed with increasing sample temperature. However, as was previously demonstrated by the power-dependence measurements, no significant spectral shift was observed. This raises a
question regarding the true temperature of the emitting sample. The temperature of the emitting graphene ceramics was measured using the Er3+-doped LiYb0.99Er0.01P4O12 upconverting
nanocrystalline sensors encrusted on the surface of the emitting sample. The temperature sensing was achieved based on the well-known phenomenon that the ratio of the 4S3/2→4I15/2 and
2H11/2→4I15/2 transitions is related to the change in temperature. The emission intensity from the higher-lying state 2H11/2 depends on its population, which increases with temperature
following the Boltzmann relation (Equation (3)): where _A_ and _B_ are proportionality parameters, Δ_E_ is the energy difference between the indicated states, _T_ is the temperature and _k_B
is the Boltzmann constant. The energy gap between the states in the case of LiYb0.99Er0.01P4O12 is approximately 810 cm−1. The sample to be measured was placed in vacuum (5×10−3 hPa), and
the upconversion emission spectra were recorded (Figure 5a). Because the logarithm of the emission intensity ratio of 4S3/2→4I15/2 to 2H11/2→4I15/2 is a linear function of temperature, by
using the Boltzmann law, one is able to determine the temperature of nanocrystals subjected to laser excitation. Figure 5a presents the upconversion emission spectra of the
LiYb0.99Er0.01P4O12 nanocrystals encrusted on the graphene ceramic surface. Three characteristic _f_–_f_ fluorescence transitions of Er3+ located within the broadband WL emission of the
graphene ceramics are clearly observed. For the low-optical-power regime, only the Er3+ fluorescence signatures localized near approximately 520 nm, 540 nm and 650 nm were observed; these
signatures are associated with the 2H11/2→4I15/2, 4S3/2→4I15/2 and 4F9/2→4I15/2 transitions, respectively. With an increase in excitation power, the intensities of the UC transitions also
increased. The results revealed that even for a very high excitation power of 1.5 W at _λ_exc=975 nm, the temperature of the sample remained lower than 900 K, and for excitation powers lower
than 1 W, the temperature was lower than 700 K. To determine whether the WL emission from the graphene ceramics could also be observed at lower temperatures, additional experiments were
performed in a closed-cycle cryostat. The results are presented in Figure 5b. No particular temperature dependence was observed; indeed, the WL emission intensity remained nearly constant
across a wide range of temperatures, from 10 K to 300 K. It should be noted that similar experiments performed to investigate the anti-Stokes WL emission induced by an infrared laser in a
dielectric host in the form of LiNdP4O12 nanocrystals22 have demonstrated clear evidence of temperature dependence, with complete quenching of the emission at very low temperatures. These
results provide a very compelling reason to reconsider the interpretation of the origins of laser-induced WL emission from graphene. The photoelectrical response of the graphene ceramics
provides additional information regarding the origin of the WL emission. The time-dependent photoelectric response of the graphene ceramics for 975-nm laser excitation at various powers is
presented in Figure 6a. A linear dependence of the change in resistivity Δ_ρ_ on the excitation power was observed, with values up to Δ_ρ_≈0.5 mΩ cm. The time-dependent characteristics of
the process for two representative wavelengths of _λ_exc=405 nm and 975 nm are depicted in Figure 6b. The photo-effect associated with the interband transition is pronounced for 405 nm
excitation; however, thermo-electric effects contribute to the process, as is observed from the long saturation tail. The thermopower values of graphene ceramics may differ among samples,
depending on their preparation temperatures; at 300 K, these values may change from 6 µV K−1 to 20 µV K−1. A curve with a somewhat different character was observed for the 975-nm excitation
wavelength. Pronounced effects of thermal processes were also clearly observed. None of the curves was observed to follow the first-order rate law, implying that more than one process was
involved in the change in resistivity. The time constants of the processes under 975-nm excitation were estimated to be _τ_1=10.8 s and _τ_2=63.2 s. There are several considerations that may
be useful in forming any conclusions to be drawn regarding the origin of the WL emission from graphene ceramics. Because pristine graphene has no energy gap, no radiative emission is
observed under typical conditions of optical excitation. On the other hand, emission from thermalized electrons—incandescence—requires temperatures much higher than those measured in this
work. It was also demonstrated that the WL emission is a threshold process, which suggests that emission from graphene may be observed after a certain energy barrier is crossed (in our
experiments, _E_tot=_N_exc_ħ_ωexc≈8 eV), leading to the creation of transient, non-equilibrium phases with a different distribution of charge and a different electronic structure. It has
previously been demonstrated that non-equilibrium states in graphene involving a population of inverted dense Dirac fermions emerge during femtosecond pulse propagation.25 This observation
supports the concept of photoinduced transitions of sp2→sp3 hybridization in disordered phases such as graphene ceramics. Several studies have been published concerning light emission under
femtosecond light stimulation.26 However, the first reports of CW-excited hot luminescence were published by Chen _et al_.,27 who were studying inelastic light scattering in highly doped
graphene; WL emission from cold samples has never previously been reported. It is also worth noting that photoinduced phase transitions involving switching between anti-ferromagnetic and
magnetic states induced by femtosecond pulses _via_ strongly correlated spin-charge quantum excitation have also been demonstrated by Li _et al_.28 in non-carbonaceous compounds
(Pr0.7Ca0.3MnO3). Previous attempts to open a band gap in graphene have concentrated on the physical modification of the material, in which delocalization of _π_ bonds induces the creation
of the band gap.29,30 In this work, we propose a model of photoinduced phase transformation to explain the WL emission from CW-excited graphene ceramics.31 The photoinduced transition
process relies on a hidden multistability of the material, meaning that in addition to the true ground state, there are also hidden, so-called false ground states that are inaccessible
through thermal fluctuations but achievable through electronic excitation. A conceptual diagram of the photoinduced transition process is presented in Figure 7. Recent theoretical32,33,34,35
and experimental36,37 results have indicated the existence of a new stable phase of carbon with mixed sp2 and sp3 hybridized bonds—diaphite. In one such experiment, highly oriented
pyrolytic graphite was excited using a femtosecond laser (1.57-eV photon energy), leading to electron transfer between the _π_ and _π_* bands. Similar effects may also be induced _via_ CW
excitation, with two or three photons inducing a charge transfer from the _π_ band to the _π_* band and creating an electron–hole pair.35,38 A conceptual diagram of this mechanism is
presented in Figure 8. The latter process leads to the creation of transient localized states of electron-hole pairs. The electron-optical phonon coupling leads to graphene lattice
contraction, whereas unlocalized states contribute to the conduction band. Henceforth, a small amount of electronic energy is converted into kinetic energy of the lattice, leading to the
heating of the material. The overall process is a multiphoton process; however, as shown in Refs. 34, 39 and 40, a minimal diaphite domain may be created when the energy deposited in the
system exceeds 4.5 eV, which corresponds to only a few visible photons. The transformation is nonlinear because of the strong coupling between neighboring atoms in graphite sheets. It is
worth noting that the resulting new phase—diaphite—has already been observed to be a quasistable structure, with stability of up to a few days.36 As a result of the sp2→sp3 transition, a
band gap opens, resulting in the creation of a semiconducting phase immersed in the semimetallic continuum. Although this process has been observed in graphite, the theoretical
interpretation presented suggests that similar behavior should occur in all carbon systems with a linear band shape in the visible energy regime. Henceforth, the observation of phenomena of
this type is to be expected in bi- and trilayer graphene, as well. Our analysis suggests that the observed emission is purely electronic in nature. This conclusion explains both the heating
of the material (energy transfer _via_ free photo-generated carrier-phonon coupling) and the power-law dependence (threshold energy required for diaphite domain formation). The remaining
majority of unlocalized carriers will contribute to the ionization process. As stated above, CW excitation will lead to two types of carriers—localized and unlocalized. Coulomb repulsion
will induce rapid separation from the excited domain to the metallic continuum (Figure 8). As a result, the remaining carbon atoms will have a lower ionization potential because of the
appearance of excess charge in the system and _π_-bond expansion. Because the ionization potential in graphene is approximately 8 eV, multiphoton direct ionization will occur. Photoinduced
carriers will recombine with ionized carbons or contribute to the conduction _π_ band, thereby lowering the ionization potential. CONCLUSIONS Laser-induced WL emission from graphene ceramics
was investigated in this work. Intense WL emission centered at 650 nm and consisting of a number of subbands was observed. No significant dependence of the broadband WL emission maximum on
the excitation wavelength was observed. Laser-induced WL emission was observed at a number of different excitation wavelengths, from the VIS to the NIR range, i.e., at 476 nm, 488 nm, 514
nm, 808 nm and 974 nm. It was also observed that the WL emission from graphene ceramics was a threshold process exhibiting supralinear behavior. It was observed that the intensity of the
laser-induced WL emission increased nonlinearly with increasing incident laser power, with a strong increase in intensity after a certain power threshold that could be well fitted using a
power law, _P__N_. The order of the process, _N_, decreased with increasing frequency of the incident laser light. Similarly, the excitation power threshold decreased with increasing
frequency of the incident laser light. The total energy _E_tot of _N_ absorbed photons with energy _ħ_ωexc remained nearly constant for all investigated excitation wavelengths,
_E_tot=_N_exc_ħ_ωexc≈8 eV. It was also demonstrated that the intensity of the WL emission was highly dependent on the ambient pressure. The highest intensity was observed at the lowest
pressure. The temperatures of the samples were also measured using LiYb0.99Er0.01P4O12 upconverting nanocrystals encrusted on the surfaces of the graphene ceramics. The results revealed that
even for a very high excitation power of 1.5 W at _λ_=975 nm, the temperature of the sample remained lower than 900 K, and for excitation powers lower than 1 W, the temperature was below
700 K. No dependence of the WL emission intensity on the sample temperature was observed, and WL emission was also observed at cryogenic temperatures as low as 10 K. This result provides
compelling evidence that even when the sample is hot, its temperature is still far too low to explain the emission according to the theory of thermalized electrons. Efficient photocurrent
was observed in the graphene ceramics. It increased linearly with increasing power of the incident laser light. A model of photoinduced, transient, domain-like sp2→sp3 phase transitions was
proposed to explain the origin of the multiphoton-induced WL emission in graphene ceramics. REFERENCES * Geim AK, Novoselov KS . The rise of graphene. _Nat Mater_ 2007; 6: 183–191. Article
ADS Google Scholar * Schwierz F . Graphene transistors. _Nat Nanotechnol_ 2010; 5: 487–496. Article ADS Google Scholar * Chen MT, Tao T, Zhang L, Gao W, Li CZ . Highly conductive and
stretchable polymer composites based on graphene/MWCNT network. _Chem Commun_ 2013; 49: 1612–1614. Article Google Scholar * Liu YX, Dong XC, Chen P . Biological and chemical sensors based
on graphene materials. _Chem Soc Rev_ 2012; 41: 2283–2307. Article Google Scholar * He QY, Wu SX, Yin ZY, Zhang H . Graphene-based electronic sensors. _Chem Sci_ 2012; 3: 1764–1772.
Article Google Scholar * Loh KP, Bao QL, Eda G, Chhowalla M . Graphene oxide as a chemically tunable platform for optical applications. _Nat Chem_ 2010; 2: 1015–1024. Article Google
Scholar * Li M, Cushing SK, Zhou XJ, Guo SW, Wu NQ . Fingerprinting photoluminescence of functional groups in graphene oxide. _J Mater Chem_ 2012; 22: 23374–23379. Article Google Scholar
* de Volder MF, Tawfick SH, Baughman RH, Hart AJ . Carbon nanotubes: present and future commercial applications. _Science_ 2013; 339: 535–539. Article ADS Google Scholar * Dresselhaus MS,
Dresselhaus G . Fullerenes and fullerene-derived solids as electronic materials. _Annu Rev Mater Sci_ 1995; 25: 487–523. Article ADS Google Scholar * Guldi DM, Illescas BM, Atienza CM,
Wielopolski M, Martín N . Fullerene for organic electronics. _Chem Soc Rev_ 2009; 38: 1587–1597. Article Google Scholar * Novoselov KS, Fal’ko VI, Colombo L, Gellert PR, Schwab MG _et al_.
A roadmap for graphene. _Nature_ 2012; 490: 192–200. Article ADS Google Scholar * Castro Neto AH, Guinea F, Peres NM, Novoselov KS, Geim AK . The electronic properties of graphene. _Rev
Mod Phys_ 2009; 81: 109–162. Article ADS Google Scholar * Yu DS, Dai LM . Voltage-induced incandescent light emission from large-area graphene films. _Appl Phys Lett_ 2010; 96: 143107.
Article ADS Google Scholar * Scarselli M, Castrucci P, de Crescenzi M . Electronic and optoelectronic nano-devices based on carbon nanotubes. _J Phys Condens Mat_ 2012; 24: 313202.
Article ADS Google Scholar * Mak KF, Ju L, Wang F, Heinz TF . Optical spectroscopy of graphene: from the far infrared to the ultraviolet. _Solid State Commun_ 2012; 152: 1341–1349.
Article ADS Google Scholar * Ferrari AC, Meyer JC, Scardaci V, Casiraghi C, Lazzeri M _et al_. Raman spectrum of graphene and graphene layers. _Phys Rev Lett_ 2006; 97: 187401. Article
ADS Google Scholar * Ikezawa S, Wakamatsu M, Ueda T . Optical analytical technique for carbonaceous particles using laser-induced electro-avalanche fluorescence and laser-induced
incandescence. _Sensor Mater_ 2013; 25: 57–77. Google Scholar * Essig S, Marquardt CW, Vijayaraghavan A, Ganzhorn M, Dehm S _et al_. Phonon-assisted electroluminescence from metallic carbon
nanotubes and graphene. _Nano Lett_ 2010; 10: 1589–1594. Article ADS Google Scholar * Biswas C, Güneş F, Loc DD, Lim SC, Jeong MS _et al_. Negative and positive persistent
photoconductance in graphene. _Nano Lett_ 2011; 11: 4682–4687. Article ADS Google Scholar * Dong YQ, Shao JW, Chen CQ, Li H, Wang RX _et al_. Blue luminescent graphene quantum dots and
graphene oxide prepared by tuning the carbonization degree of citric acid. _Carbon_ 2012; 50: 4738–4743. Article Google Scholar * Sekiya R, Uemura Y, Murakami H, Haino T .
White-light-emitting edge-functionalized graphene quantum dots. _Angew Chem Int Ed_ 2014; 53: 5619–5623. Article Google Scholar * Strek W, Marciniak L, Gluchowski P, Hreniak D . Infrared
laser stimulated broadband white emission of Yb3+:YAG nanoceramics. _Opt Mater_ 2013; 35: 2013–2017. Article ADS Google Scholar * Strek W, Marciniak L, Bednarkiewicz A, Lukowiak A,
Wiglusz R _et al_. White emission of lithium ytterbium tetraphosphate nanocrystals. _Opt Express_ 2011; 19: 14083–14092. Article ADS Google Scholar * Wang JW, Tanner PA . Upconversion for
white light generation by a single compound. _J Am Chem Soc_ 2010; 132: 947−949. Article Google Scholar * Li T, Luo L, Hupalo M, Zhang J, Tringides MC _et al_. Femtosecond population
inversion and stimulated emission of dense Dirac fermions in graphene. _Phys Rev Lett_ 2012; 108: 167401. Article ADS Google Scholar * Lui CH, Mak KF, Shan J, Heinz TF . Ultrafast
photoluminescence from graphene. _Phys Rev Lett_ 2010; 105: 127404. Article ADS Google Scholar * Chen CF, Park CH, Boudouris BW, Horng J, Geng B _et al_. Controlling inelastic light
scattering quantum pathways in graphene. _Nature_ 2011; 471: 617–620. Article ADS Google Scholar * Li T, Patz A, Mouchliadis L, Yan J, Lograsso TA _et al_. Femtosecond switching of
magnetism _via_ strongly correlated spin-charge quantum excitations. _Nature_ 2013; 496: 69–73. Article ADS Google Scholar * Han MY, Özyilmaz B, Zhang YB, Kim P . Energy band-gap
engineering of graphene nanoribbons. _Phys Rev Lett_ 2007; 98: 206805. Article ADS Google Scholar * Shen Y, Yang SB, Zhou P, Sun QQ, Wang PF _et al_. Evolution of the band-gap and optical
properties of graphene oxide with controllable reduction level. _Carbon_ 2013; 62: 157–164. Article Google Scholar * Huai P, Nasu K . Theory of photoinduced phase transition in the
quasi-one-dimensional charge transfer compound TTF-CA. _Int J Mod Phys B_ 2001; 15: 3714–3717. Article ADS Google Scholar * Ohnishi H, Nasu K . Photoinduced domain-type collective
structural changes with interlayer sigma-bonds in the visible region of graphite. _Phys Rev B_ 2009; 79: 054111. Article ADS Google Scholar * Ohnishi H, Nasu K . Generation and growth of
_sp_3 bonded domains by visible photon irradiation of graphite. _Phys Rev B_ 2009; 80: 014112. Article ADS Google Scholar * Nishioka K, Nasu K . Early-stage real-time dynamics of
interlayer _sp_3 bond formation by visible-light irradiation of graphite. _Phys Rev B_ 2009; 80: 235420. Article ADS Google Scholar * Radosinski L, Nasu K, Luty T, Radosz A . Possible
domain-type collective dimerization of graphite induced by interlayer charge transfer excitations in the visible region. _Phys Rev B_ 2010; 81: 035417. Article ADS Google Scholar *
Kanasaki J, Inami E, Tanimura K, Ohnishi H, Nasu K . Formation of _sp_3 bonded carbon nanostructures by femtosecond laser excitation of graphite. _Phys Rev Lett_ 2009; 102: 087402. Article
ADS Google Scholar * Raman RK, Murooka Y, Ruan CY, Yang T, Berber S _et al_. Direct observation of optically induced transient structures in graphite using ultrafast electron
crystallography. _Phys Rev Lett_ 2008; 101: 077401. Article ADS Google Scholar * Radosinski L, Ostasiewicz K, Luty T, Radosz A, Nasu K . Domain-type collective dimerization of graphite
and possible _sp_2→_sp_3 transition induced by inter-layer charge transfer excitations in the visible region. _Acta Phys Pol A_ 2010; 118: 500–506. Article Google Scholar * Radosinski L,
Luty T, Nasub K, Ohnishi H, Nishioka K _et al_. Photoinduced conversion of hybridization in graphite. _Acta Phys Pol A_ 2012; 121: 359–368. Article Google Scholar * Nishioka K, Nasu K .
Cooperative domain-type interlayer _sp_3 bond formation in graphite. _Phys Rev B_ 2010; 82: 035440. Article ADS Google Scholar Download references ACKNOWLEDGEMENTS This work was supported
by Wroclaw Research Centre EIT+ within the framework of the project ‘The Application of Nanotechnology in Advanced Materials’—NanoMat (POIG.01.01.02-02-002/08) financed by the European
Regional Development Fund (Operational Programme Innovative Economy, 1.1.2). _NOTE: ACCEPTED ARTICLE PREVIEW ONLINE 19 NOVEMBER 2014_ AUTHOR INFORMATION AUTHORS AND AFFILIATIONS * Polish
Academy of Sciences, Institute of Low Temperatures and Structural Research, 50-422 Wroclaw, Poland Wieslaw Strek, Bartlomiej Cichy, Pawel Gluchowski, Lukasz Marciniak, Mikolaj Lukaszewicz
& Dariusz Hreniak * Wroclaw University of Technology, Group of Chemical and Biochemical Processes, 50-370 Wroclaw, Poland Lukasz Radosinski Authors * Wieslaw Strek View author
publications You can also search for this author inPubMed Google Scholar * Bartlomiej Cichy View author publications You can also search for this author inPubMed Google Scholar * Lukasz
Radosinski View author publications You can also search for this author inPubMed Google Scholar * Pawel Gluchowski View author publications You can also search for this author inPubMed
Google Scholar * Lukasz Marciniak View author publications You can also search for this author inPubMed Google Scholar * Mikolaj Lukaszewicz View author publications You can also search for
this author inPubMed Google Scholar * Dariusz Hreniak View author publications You can also search for this author inPubMed Google Scholar CORRESPONDING AUTHOR Correspondence to Bartlomiej
Cichy. ADDITIONAL INFORMATION Note: Supplementary information for this article can be found on the _Light: Science & Applications'_ website. SUPPLEMENTARY INFORMATION SUPPLEMENTARY
INFORMATION (DOCX 8789 KB) RIGHTS AND PERMISSIONS This work is licensed under a Creative Commons Attribution-NonCommercial-ShareAlike 3.0 Unported License. The images or other third party
material in this article are included in the article's Creative Commons license, unless indicated otherwise in the credit line; if the material is not included under the Creative
Commons license, users will need to obtain permission from the license holder to reproduce the material. To view a copy of this license, visit
http://creativecommons.org/licenses/by-nc-sa/3.0/ Reprints and permissions ABOUT THIS ARTICLE CITE THIS ARTICLE Strek, W., Cichy, B., Radosinski, L. _et al._ Laser-induced white-light
emission from graphene ceramics–opening a band gap in graphene. _Light Sci Appl_ 4, e237 (2015). https://doi.org/10.1038/lsa.2015.10 Download citation * Received: 16 July 2014 * Revised: 31
October 2014 * Accepted: 04 November 2014 * Published: 16 January 2015 * Issue Date: January 2015 * DOI: https://doi.org/10.1038/lsa.2015.10 SHARE THIS ARTICLE Anyone you share the following
link with will be able to read this content: Get shareable link Sorry, a shareable link is not currently available for this article. Copy to clipboard Provided by the Springer Nature
SharedIt content-sharing initiative KEYWORDS * ceramics * graphene * photoluminescence * white light